Abstract
Aim:
This study aimed to examine the amount of surface non-specific adsorption, or fouling, observed by Pseudomonas aeruginosa (P. aeruginosa) on a quartz crystal based acoustic wave biosensor under different flow conditions with and without an anti-fouling layer.
Methods:
An electromagnetic piezoelectric acoustic sensor (EMPAS) based on electrode free quartz crystals was used to perform the analysis. Phosphate buffered saline (PBS) was flowed over the crystal surface at various flow rates from 50 μL/min to 200 μL/min, with measurements being taken at the 43rd harmonic (~864 MHz). The crystal was either unmodified, or modified with a monoethylene glycol [2-(3-silylpropyloxy)-hydroxy-ethyl (MEG-OH)] anti-fouling layer. Overnight culture of P. aeruginosa PAO1 (PAO1) in lysogeny broth (LB) was injected into the system, and flow maintained for 30 min.
Results:
The frequency change of the EMPAS crystal after injection of bacteria into the system was found to change based on the flow rate of buffer, suggesting the flow rate has a strong effect on the level of non-specific adsorption. The MEG-OH layer drastically reduced the level of fouling observed under all flow conditions, as well as reduced the amount of variation between experiments. Flow rates of 150 μL/min or higher were found to best reduce the level of fouling observed as well as experimental variation.
Conclusions:
The MEG-OH anti-fouling layer is important for accurate and reproducible biosensing measurements due to the reduced fouling and variation during experiments. Additionally, a flow rate of 150 μL/min may prove better for measurement compared to the current standard of 50 μL/min for this type of instrument.
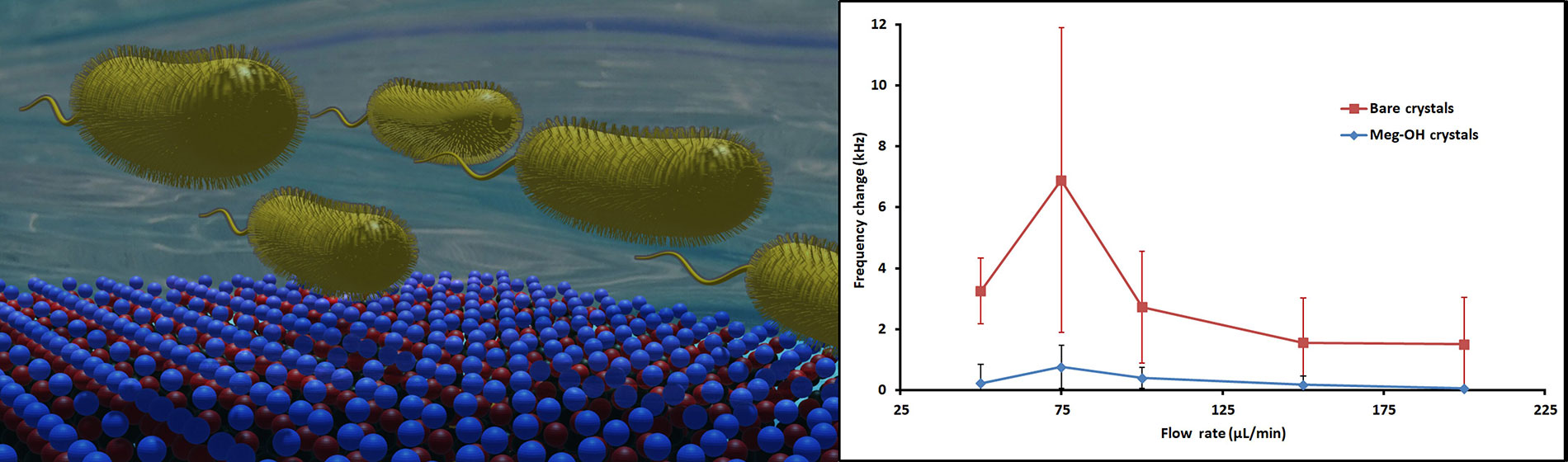
P. aeruginosa interaction with a MEG-OH surface coating on a high frequency biosensor results in a drastic reduction in observed fouling
Keywords
Anti-fouling, biosensor, acoustic wave, Pseudomonas aeruginosa , flow ratesIntroduction
Infections associated with the formation of biofilms or bio-fouling caused initially by adhesion of bacteria to the surfaces of materials employed for medical devices constitutes a serious problem in terms of patient treatment. This is especially the case for polymer-based catheters used for the treatment of urinary retention, where such infections can lead to not only severe medical complications and death, but also to significant economic cost in terms of treatment [1–4]. The initial interaction of microbes with material surfaces involves an array of interfacial factors such as charge, surface free energy, topography and the effect of hydrodynamic flow [5].
Previous research has investigated aspects of the initial interaction of bacteria with surfaces through biosensor technology. This approach offers significant potential for evaluation of the strongly-associated area of non-specific adsorption or fouling including by biochemical species. With respect to analytical chemistry, the reduction of non-specific adsorption in biosensor research is of critical importance to achieve a signal that is sensitive and selective to the analyte of interest. Many efforts have been made to develop surfaces that resist non-specific adsorption, or fouling, such as using poly-ethylene glycol, polymer brushes, and peptides [6]. Patterned surfaces have also been explored, though these surfaces are difficult to implement in many biosensors due to the need for specific topographies of the biosensing surfaces [7].
The device employed in the present work is the electromagnetic piezoelectric acoustic sensor (EMPAS), which belongs to a general class of such acoustic sensors which includes so-called quartz crystal microbalances, and surface acoustic wave (SAW) sensors. The EMPAS system was first developed in the early 2000s in order to perform acoustic wave measurements at ultra-high frequencies in the several hundred MHz [8]. This system has been extensively studied with regards to anti-fouling coatings on silica using trichlorosilanes-based layers as a method of surface adhesion [9, 10]. More recent work has involved updating the EMPAS system to operate on more modern computer hardware making it easier to operate (Figure 1) [11].
Of the anti-fouling layers studied with the EMPAS system, hydroxyl terminated monoethylene glycol [2-(3-silylpropyloxy)-hydroxy-ethyl (MEG-OH)], which is added to surfaces as a trifluoroacetate protected version [2-(3-trichlorosilylpropyloxy)-ethyl trifluoroacetate (MEG-TFA)], proved to be the best at preventing surface adhesion from serum (Figure 2) [9]. This coating has been further studied on additional surfaces such as plastic polymers to prevent blood clotting [12–14], on steel to prevent blood clotting [15], and on polymers to reduce bacterial adhesion [16, 17]. Typically, EMPAS studies whether in detection or fouling have used a flow rate of 50 μL/min in order to provide interaction time between any surface probes and the analyte of interest [11, 18, 19]. However, a flow rate of 50 μL/min may also contribute to a larger amount of non-specific adsorption due to the greater amount of time the sample has to interact with the surface compared to faster flow rates.
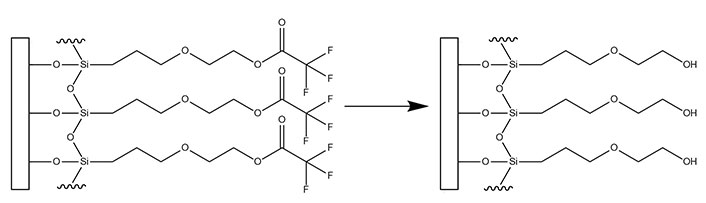
Transformation of surface bound MEG-TFA to MEG-OH
Note. Adapted from “Detection of E. Coli bacteria in milk by an acoustic wave aptasensor with an anti-fouling coating,” by Spagnolo S, De La Franier B, Davoudian K, Hianik T, Thompson M. Sensors (Basel). 2022;22:1853 (https://www.mdpi.com/1424-8220/22/5/1853). CC BY.
Considerable interest in the past has been shown in the interaction of Pseudomonas aeruginosa (P. aeruginosa) with surfaces [20–22]. In the present study, our previously-described work on bacterial fouling is combined with EMPAS measurements in order to determine the level of adhesion of P. aeruginosa that occurs on a silica (quartz) surface under different flow rates. Experiments were performed against bare EMPAS crystals, and MEG-OH coated crystals, with the expectation that MEG-OH would reduce the amount of non-specific adsorption observed at all flow rates, and that faster flow rates would contribute to lower amounts of fouling.
Materials and methods
Materials
MEG-TFA was synthesized according to previously published methods [9]. Anhydrous toluene, sodium dodecyl sulfate, sulfuric acid, 30% hydrogen peroxide (H2O2), Na2HPO4, and NaCl were purchased from Sigma-Aldrich (St. Louis, MO, USA). Ethanol and acetone were obtained from Caledon Laboratory Chemicals (Georgetown, ON, Canada). All chemicals were used without further purification. Adhesion experiments were carried out with P. aeruginosa PAO1 (PAO1) purchased from VWR Canada (Mississauga, ON, Canada). Quartz crystals (AT-cut, 13.5 mm in diameter, 20 MHz fundamental frequency) were purchased from Lap-Tech Inc., Bowmanville, Ontario.
Cleaning and modification of quartz crystals
AT-cut quartz crystals were first rinsed with distilled water, and then sonicated in a 1% solution of sodium dodecyl sulfate in distilled water (5 min). The crystals were then rinsed again with distilled water, followed by rinsing with 95% ethanol. They were then sonicated in 95% ethanol (5 min), followed by rinsing with 95% ethanol. The same was then done with acetone, followed by rinsing with distilled water to remove any leftover organic solvent.
The crystals were then submerged in piranha solution (3:1 sulfuric acid:30% H2O2) and held at 95°C using a water bath for 30 min. The crystals were then removed from the piranha solution and rinsed copiously with distilled water. They were then dried under a stream of nitrogen gas, and placed in an oven held at 185°C for 1 h to complete the drying. They were then subjected to plasma cleaning (10 min, atmospheric plasma) in order to further clean and hydroxylate the surface. Crystals for bare experiments were then stored under atmosphere at room temperature, while those for MEG-OH experiments were placed in a humidity chamber (70% relative humidity) overnight to hydrate the surface.
Crystals for modification were then placed in pre-silanized test tubes [overnight solution of 1% trichloro(octadecyl)silane in toluene], and brought into a glovebox under inert nitrogen atmosphere. A MEG-TFA solution was prepared (1:1,000 MEG-TFA:anhydrous toluene) and added to each crystal (2 mL/crystal). The tubes were sealed and removed from the glovebox and placed on a rotator at 120 rpm for 90 min. After this the crystals were rinsed with toluene, sonicated in toluene (5 min), and further rinsed with toluene. They were then rinsed with 95% ethanol to remove any remaining toluene, before being deprotected overnight in a 50% ethanol solution under rotation (120 rpm). The crystals were then rinsed with 95% ethanol, followed by acetone, and dried under nitrogen to yield MEG-OH coated crystals.
EMPAS experiments
EMPAS experiments were carried out using similar methods to those previously described [11]. The syringe pump was operated in pushing mode, pushing a solution of phosphate buffered saline [PBS; 10 mmol/L Na2HPO4, 154 mmol/L NaCl, pH 7.4] though an Eppendorf auto-injector, into the crystal chamber, and into a waste receptacle. A bare or MEG-OH modified crystal was placed in the custom flow-through cell, and secured using a screw clamp. The flow rate was set to between 50 μL/min and 200 μL/min in order to compare the amount of observed fouling at different flow rates. The crystal was measured at the 43rd harmonic (~864 MHz). After stable baseline was achieved a 250 μL injection loop was filled with PAO1 [overnight culture at 37°C in lysogeny broth (LB)], or LB for control, and immediately injected into the system. PBS flow was continued for 30 min following injection.
The filling tubing was then cleaned with 12% bleach (1.5 mL), followed by 95% ethanol (1.5 mL), and distilled water (1.5 mL). Air was then pulled through the filling tubing to remove any remaining water. The main tubing, injection loop, and crystal chamber were then cleaned in the same fashion, and the crystal then replaced for further experiments.
Results
Example EMPAS runs with injection of PAO1 at each flow rate are shown in Figure 3 for bare crystals (Figure 3A) and MEG-OH coated crystals (Figure 3B). The data was normalized to the baseline, and injection times lined up for clarity. The example runs that showed the closest overall change to the average for each flow rate were chosen for Figure 3. As seen in Figure 3, the frequency change following injection remained relatively stable, showing very little wash-off from continued PBS flow regardless of flow rate.
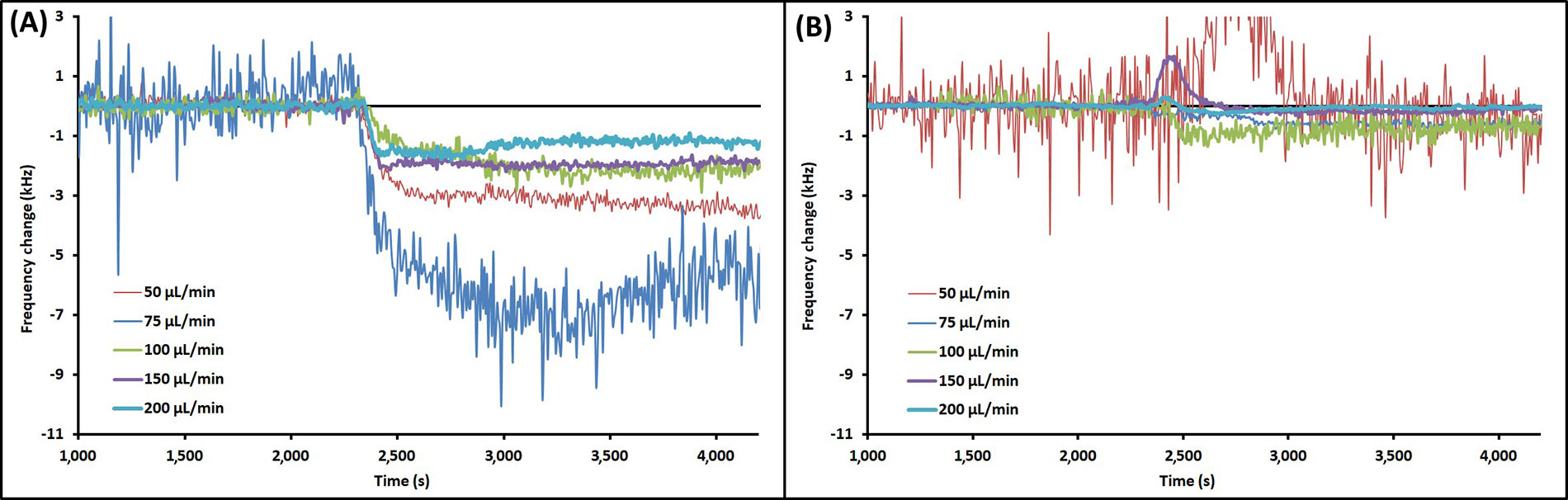
Example EMPAS runs for (A) bare quartz crystals and (B) MEG-OH coated quartz crystals at various flow rates with injection of 250 μL PAO1 in LB at ~2,300 s. Data normalized to baseline frequency
Runs were also performed with just LB, which was found to also foul bare quartz crystals, but to a lesser extent than the PAO1 overnight cultures (Figure 4A). For pure LB runs the greatest degree of frequency change was at a flow rate of 100 μL/min. As for MEG-OH coated crystals although some frequency shift was observed for some flow rates, the frequency returned to baseline within the 30 min of PBS wash-off (Figure 4B).
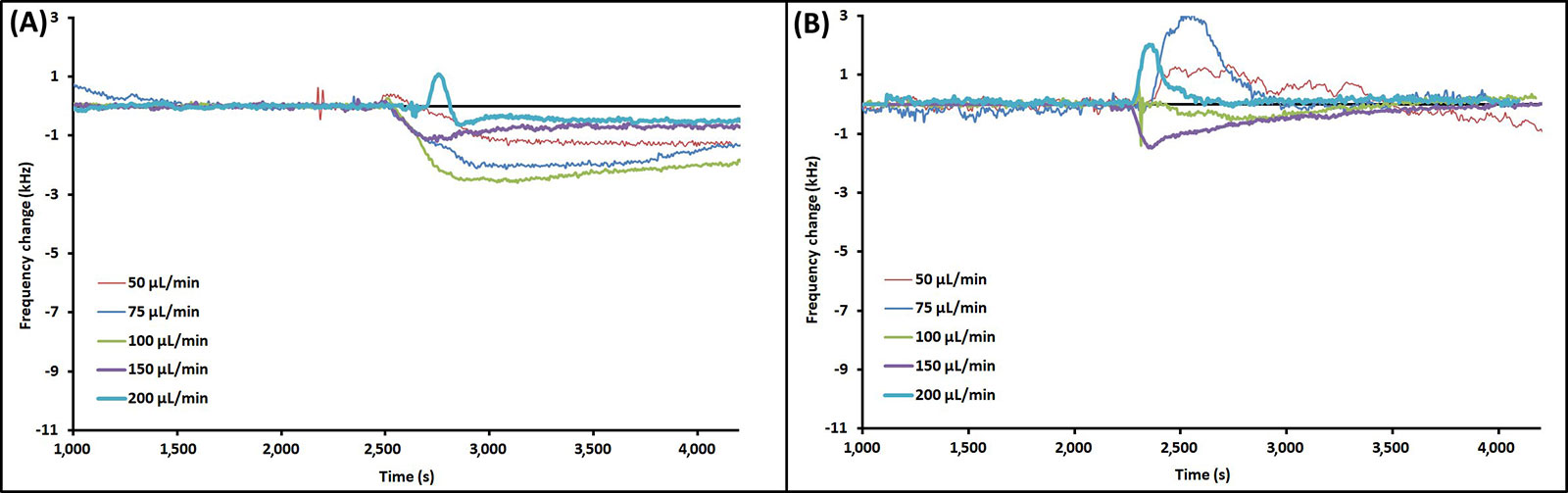
Example EMPAS runs for (A) bare quartz crystals and (B) MEG-OH coated quartz crystals at various flow rates with injection of 250 μL LB at ~2,300 s. Data normalized to baseline frequency
Several runs were performed for each flow rate, and the change in frequency was calculated from average frequency prior to injection, to the average frequency 30 min after injection. The averages for these runs are shown in Table 1, as well as the percent reduction in frequency change as a result of the MEG-OH surface coating. As can be seen MEG-OH reduced the amount of frequency change observed, and thus the amount of fouling caused by PAO1. Oddly the highest change in frequency occurred at a flow rate of 75 μL/min, with the slower flow rate of 50 μL/min showing a lower amount of fouling. Faster flow rates show the expected trend of reduced fouling as the flow rate is increased, with diminishing returns observed above a flow rate of 150 μL/min. The data from Table 1 is visualized in Figure 5.
Average frequency changes 30 min after injection of 250 μL PAO1 over bare and MEG-OH coated crystals at various flow rates, and the percent reduction in frequency change caused by the MEG-OH coating
Flow rate (μL/min) | Bare crystal ΔFrequency (kHz) | MEG-OH crystal ΔFrequency (kHz) | Reduction in change (%) |
---|---|---|---|
50 | 3.25 ± 1.08 | 0.22 ± 0.63 | 93% |
75 | 6.89 ± 5.00 | 0.75 ± 0.70 | 89% |
100 | 2.72 ± 1.83 | 0.40 ± 0.34 | 85% |
150 | 1.56 ± 1.47 | 0.18 ± 0.28 | 89% |
200 | 1.50 ± 1.55 | 0.05 ± 0.05 | 97% |
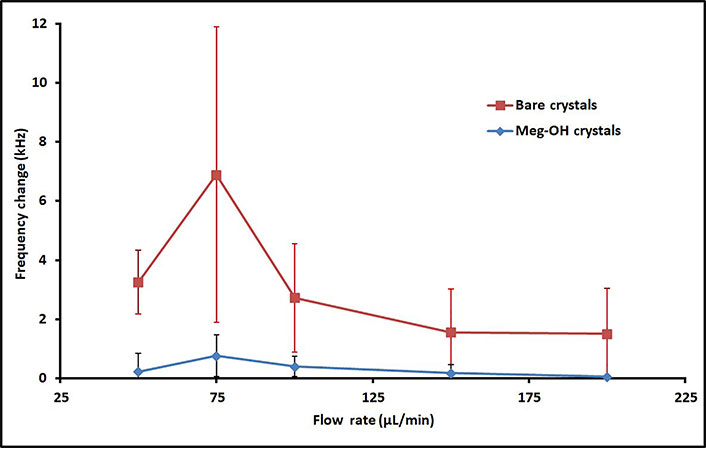
Average frequency changes 30 min after injection of 250 μL PAO1 over bare (red line) and MEG-OH coated (blue line) crystals at various flow rates
Discussion
As can be seen from the change in frequency, PAO1 is able to foul the surface of bare quartz with ease during the brief interaction time of an experiment. Between a flow rate of 50 μL/min and 200 μL/min the change in frequency generally decreases as the flow rate increases. This makes intuitive sense, as the higher the flow rate the less time the bacteria has to interact with the surface, and the more shear force is present reducing adhesion. Interestingly there is a sharp rise in frequency change at 75 μL/min observed for both bare and MEG-OH coated crystals, setting that flow rate apart from the trend. This may be due to increased fouling of the injection tubing at the slower 50 μL/min flow rate, reducing the amount of bacteria that reaches the surface at that flow rate, as opposed to a greater ability of the bacteria to adhere to the surface of the crystal at the higher 75 μL/min flow rate. However, more work would need to be done to determine the exact cause of this rise in fouling observed at the 75 μL/min flow rate.
At all flow rates the magnitude of frequency change was reduced by the presence of MEG-OH on the crystal surface. At its lowest this reduction in observed change was 85% at a 100 μL/min flow rate, while it provided the greatest degree of reduction at a flow rate of 200 μL/min with a 97% reduction. This suggests that regardless of the flow rate MEG-OH is able to drastically hinder the ability of PAO1 to adhere to the surface under flow. This is in agreement with previous research, which found an 85% reduction in PAO1 fouling of MEG-OH coated polyurethane compared to uncoated polyurethane under static conditions [16].
When only LB was injected over the crystal there was also some frequency change observed, suggesting some fouling as a result of the LB itself. This shift was less than the shift observed for PAO1 overnight culture, typically less than a third of the amount, suggesting that even though LB does contribute somewhat to the observed fouling, the bacteria itself is fouling the surface to a greater extent. MEG-OH coated crystals showed no lasting frequency change when exposed to LB, with any shift in frequency caused by injection returning to baseline within the 30 min of PBS flow. This suggests that MEG-OH is able to prevent any observable fouling from LB itself, and that the observed shift seen during experiments was a result of PAO1 itself fouling the surface. Even factoring this in the frequency shift reduction from bare crystals to MEG-OH coated crystals after exposure to PAO1 was far greater than the shift observed for LB on bare crystals for all flow rates, thus MEG-OH did still reduce PAO1 fouling of the surface itself.
Another thing to note with regards to the frequency change observed for these experiments is the very high variation. For most flow rates the standard deviation of measurements is almost as high as the average frequency change. This is most likely due to the non-specific nature of these measurements; with no probe to specifically bind an analyte of interest, the amount of material, in this case bacteria, that binds to the surface is somewhat random as confirmed by this high variation. Since MEG-OH coatings reduce the change in observed frequency, they also reduce the observed variation between measurements. This is extremely important to biosensing applications, as consistent signal changes are key to accurately measuring ones analyte of interest. Anti-fouling coatings should thus be a standard in any biosensing applications, with MEG-OH proving to be a very capable coating.
As discussed in the introduction, a flow rate of 50 μL/min has been the standard for measurement using the EMPAS system. However, this research indicates that it may not be optimal for reducing the background signal that results from surface fouling. For bare crystals only the 75 μL/min flow rate showed greater fouling and variation between measurements, with the 100 μL/min flow rate being very similar and only reduced to a reasonable degree at 150 μL/min and 200 μL/min flow rates, though the high variation reduces the significance of this reduction. With a MEG-OH anti-fouling coating present a flow rate of 150 μL/min showed far less variation and a slightly lower average change in frequency compared to the standard 50 μL/min, and 200 μL/min greater still. This suggests that a flow rate of 150 μL/min or more will help reduce observed surface fouling and reduce the variation in signal change for experiments. With biosensing experiments this has to be weighed against probe and analyte binding, and the necessary interaction time for that to occur, but a higher flow rate is worth considering for any such experiments.
Further work in this area should focus on investigating other bacteria and microbes, and their ability to foul EMPAS crystals during experiments under different flow rates. Additionally, a biosensor for sensing bacteria based on the EMPAS system, likely using an aptamer as a surface probe, should be tested under different flow rates to determine if higher flow rates are able to improve measurement signals without compromising the ability of the probe to bind to the bacteria.
Abbreviations
EMPAS: |
electromagnetic piezoelectric acoustic sensor |
LB: |
lysogeny broth |
MEG-OH: |
2-(3-silylpropyloxy)-hydroxy-ethyl |
MEG-TFA: |
2-(3-trichlorosilylpropyloxy)-ethyl trifluoroacetate |
P. aeruginosa : |
Pseudomonas aeruginosa |
PAO1: |
Pseudomonas aeruginosa PAO1 |
PBS: |
phosphate buffered saline |
Declarations
Acknowledgments
We would like to acknowledge Gabor Mészáros of the Hungarian Center for Natural Science, Budapest, and Michal Sobota, Tomáš Vincze, and Tomáš Debnár of the Slovak University in Bratislava and Powertec s.r.o. for their hard work in developing the updated EMPAS system.
Author contributions
BDLF: Conceptualization, Investigation, Writing—original draft, Writing—review & editing. MT: Writing—original draft, Writing—review & editing, Validation, Supervision. Both authors read and approved the submitted version.
Conflicts of interest
The authors declare that they have no conflicts of interest.
Ethical approval
Not applicable.
Consent to participate
Not applicable.
Consent to publication
Not applicable.
Availability of data and materials
All datasets for this study are included in the manuscript.
Funding
This research was funded in part by the
Copyright
© The Author(s) 2024.