Abstract
This review highlights the challenges of current wound healing methods, such as scar formation and limited regeneration, and emphasizes the potential of tissue engineering to address these issues. Chitosan, a biopolymer derived from chitin, has garnered significant attention in epidermal-dermal wound healing due to its exceptional biocompatibility, biodegradability, and versatile functional properties. This review article delves into the diverse roles of chitosan, with a particular focus on its use as a scaffold material with fine-tunable physicochemical and biological properties for accelerated wound healing. While bare chitosan provides a suitable microenvironment for cell adhesion and proliferation, it exhibits limited mechanical strength and drug-delivery properties. However, combining it with other natural and synthetic polymers and nanoparticles facilitates drug and biosignal delivery and enhances biocompatibility and antibacterial activity. Furthermore, the review covers various chemical modifications of chitosan, including quaternization and methacrylation, to improve biocompatibility, water solubility and mechanical strength, for developing advanced wound dressings for effective skin regeneration. The review also discusses various types of smart chitosan hydrogels and the clinical translation of chitosan based scaffolds for wound healing and tissue regeneration applications. Finally, it discusses the integration of 3D bioprinting techniques for creating complex, cell-incorporated scaffolds for advanced wound healing therapies.
Keywords
Chitosan, scaffolds, wound healing, epidermal-dermal substitutes, hydrogels, chitosan-based nanoparticlesIntroduction
The skin is a complex organ consisting of various cell types and extracellular matrix (ECM) components, to protect us from environmental threats, regulate our body temperature, and enable us to perceive sensory information. It is a multilayered tissue, constituting approximately 15% of the body’s total weight, and is the largest organ of the human body. The outer epidermis, dermis, and inner subcutaneous are the three layers of skin [1] (Figure 1).
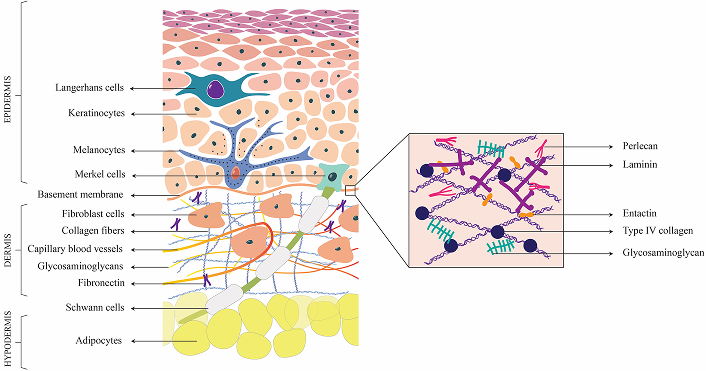
Structure of Skin. This figure represents the cross-sectional view of the skin, segmented into epidermis, dermis and hypodermis, and highlights the diverse cellular and extracellular matrix components that constitute each layer
The epidermis consists of keratinocytes and protects the body against physical, chemical, and biological insults. The epidermis also contains specialized cells, including melanocytes, Merkel cells, Langerhans cells, and various immune cells. The Merkel cells are found at the basement membrane and complexes with neurites to produce touch receptors. Melanocytes produce melanin and are also neuromodulatory and immunomodulatory in function [2]. The process of self-renewal of the epidermis is driven by epidermal stem cells located in the basal layer of the stratified squamous epithelium [3].
The dermal layer consists of columnar keratinocytes which are crucial for wound recovery and keratin production, respectively. The dermis, predominantly composed of fibroblast cells, form a highly intricate and organized architectural structure. It consists of a convoluted network of blood vessels and the lymphatic system, which facilitate nutrient and oxygen delivery, waste products and interstitial fluid elimination, and immune response [4], thereby contributing to the overall homeostasis of the skin. The fibroblast cells synthesize the ECM which forms the structural framework of tissue and supports cellular activities. The ECM of the dermal layer consist of collagen type I & III, that interact with other proteins such as laminins, nidogens, fibronectin, etc. to form a network [5]. Sweat glands, hair follicles, capillaries, immune cells, and nerves are other specialized cells present in the dermis. Inner to the dermis, hypodermis is composed of adipose tissues and connective tissues support vital functions such as cushioning and protection of deeper structures, act as an energy reservoir, protect the body from temperature fluctuations, and anchor the skin to underlying muscles and bones [5].
The basement membrane is an acellular matrix that functions to delineate and separate the epidermis from the underlying dermis, whereas the interstitial matrix is confined to interstitial spaces. Both basement membrane and interstitial matrix are rich in different collagen subtypes, which contribute to mechanical and structural properties [6]. Elastin and microfibrils that contribute to the elasticity and resilience of the skin cells are mainly found distributed in the dermis, interstitial matrix, and vessels. Fibrillin-1 & 2 are the two major classes of microfibril components of ECM. Fibronectin and laminins are the major cell adhesion glycoproteins of dermal ECM. Fibronectin is the principal component of dermis and interstitial matrix and is crucial for their interaction with collagen. This collagen-fibronectin interaction is responsible for the cellular adhesion, and migratory pathway establishment through the non-covalent crosslinking between fibronectin and collagen. Various aspects such as cellular adhesion and migration, growth and differentiation, and survival of cells are made possible through the specific interaction of these adhesive proteins with cells [7]. Stabilization of epidermal adhesion and epidermal-dermal communication is achieved through laminins.
Matricellular proteins such as thrombospondin (TSP-1), periostin, and tenascin C are dynamic regulatory proteins that contribute binding sites for various matrix molecules and cell surface receptors. Key glycosaminoglycans (GAGs) of skin ECM include hyaluronic acid, heparan, and dermatan sulfate. Hyaluronic acid/hyaluronan (HA) is mainly present in the interstitial matrix and dermal area. Dermal fibroblast cells are responsible for the secretion of dermal HA molecules and they crosslink with collagen molecules, contributing to enhanced tissue stiffness. Proteoglycans enriched with dermatan sulfate are vital components of the ECM of skin cells and primarily include decorin, biglycan, thrombomodulin and versican [8, 9].
The hierarchical arrangement of different cell types and specialized ECM components in three layers of skin makes it a unique multifunctional organ. The regenerative potential of skin is relatively good in a healthy young individual with the proliferative capability of resident cells and stem cell population. However, whenever the skin’s natural regenerative capacity is insufficient or overwhelmed in severe conditions such as burns, deep wounds, or genetic skin disorders, there should be a rapid response strategy to repair the damage and restore the skin’s functions.
Skin grafting is used to treat extensive burn injuries and involves transplanting skin from a donor, which can be autograft, allograft, xenograft, or post-mortem allograft. Being an immunocompetent organ, the clinical gold standard involves the utilization of split-thickness autologous skin grafts for treating skin defects. However, this procedure is also associated with complications such as donor site morbidity, hyperpigmentation, itching, infection, and scarring [10]. Though autologous transplantation techniques, such as Meek and mesh grafts, are available, the scarcity of skin for grafting puts a constraint on extensive skin impairment strategies [11]. The use of allografts could result in excessive scarring, tissue rejection, risk of pathogens and ultimately necessitate replacement with an autograft, albeit their vascularization within 2–3 days [12, 13]. Prolonged immunosuppression during allograft transplantation introduces other long-term risks, such as an elevated vulnerability to infections, cardiovascular disorders and cancer. Acellular skin substitutes and engineered epidermal and dermal substitutes are commercially available to treat burns, trauma, and infectious wounds [14]. Few commercially available skin substitutes, their advantages, and limitations are listed in Table 1.
List of a few commercially available skin substitutes and their properties
Sl no. | Skin substitute | Source | Characteristics | Advantages | Limitations | Ref. |
---|---|---|---|---|---|---|
1 | Alloderm SelectTM RTM | Human tissue matrix | Acellular dermal and basement membrane substitute | Intact basement membrane with appropriate dermal porosity | Not guaranteed to be free of all pathogens, high cost, long-term evaluation of carcinogenic or mutagenic potential is pending | [15–17] |
2 | Dermagraft® | Cryopreserved human fibroblasts seeded on bioabsorbable mesh | Dermal substitute used for the treatment of diabetic wounds | Treat full-thickness diabetic foot ulcers in patients who have an adequate blood supply | Absence of immune, vascular cells. Not useful for infected wounds | [15] |
3 | Glyaderm® | NaOH treated & glycerol preserved human cadaver skin | Acellular dermis is used to reconstruct full-thickness wounds arising from burns and injuries | Cost-effective, eliminate dermal antigenic structures | It is a two-step procedure, where Glyaderm application is followed by split-thickness autograft procedure | [18, 19] |
4 | IntegraTM | Dermal substitute consisting of bovine tendon collagen with chondroitin 6 sulfate with silicone surface layer | Acute deep partial-thickness, full-thickness burns, burn reconstruction applications | Availability and shelf storage, promote vascularization in poor recipient sites | Collection of hematomas and seromas is difficult. Lack of intrinsic antibacterial property | [15, 20–25] |
5 | MatriDerm® | Bovine source. Collagen-elastin hydrolysate, gamma-treated | Soft tissue defects, full thickness or deep dermal burns, and chronic wounds | Better cosmetic appearance, superior resilience, standardized option | Lack of more scientific evidence | [23, 26] |
6 | ApligrafTM | Bi-layered, prepared from neonatal foreskin-derived keratinocytes and fibroblasts with bovine type I collagen | Full-thickness diabetic wounds | Viable method of burn wound treatments, better cosmetic results, shelf-life of 5 days at room temperature | Expensive, short shelf life, instability at the wound bed | [27, 28] |
7 | Biobrane® | Inner layer of nylon mesh and an outer layer of silastic | Burn wounds (partial-thickness burns in children) | Experience less pain and requirements of pain medications, reduces time required for wound healing | Permanent scarring in partial-thickness scald wounds | [28] |
8 | Hyalomatrix® | Bilayer hyaluronan base scaffold with autologous fibroblast, and outer silicone layer present | Burn wounds and chronic wounds | Delivers hyaluronan to the wound bed | Less rigidity than some collagen-based products, risks separation with tissues under tension | [28, 29] |
9 | Transcyte® (or Dermagraft-TC) | Human fibroblast-derived, polymer membrane and newborn human fibroblast cells cultured on a porcine collagen coated nylon mesh | Licensed by the FDA for use in burns | Spontaneous separation occurs which indicates wound bed healing. Biocompatible, protects the burn wound surface from environmental insults | High cost, multiple applications required | [30–32] |
10 | Dermagraft® | Similar to Transcyte® but it lacks the silicone layer. Contains viable fibroblasts | Used in diabetic foot ulcers | Stimulate wound healing in chronic wounds | Should be used along with standard wound care regimens, useful in patients who have adequate blood supply to the involved foot | [33, 34] |
3D scaffolds for engineering of epidermal-dermal substitutes
At the clinical level, patients afflicted with severe skin injuries are treated with tissue-engineered epidermal-dermal substitutes to promote faster healing, restore skin functions, and enhance recovery outcomes. Following a skin injury, the natural healing process at the target site is hindered by the fibrous scar development due to tissue granulation and is often accompanied by limited tissue re-epithelialization. This leads to impaired nerve function and reduced skin sensitivity. Furthermore, incomplete regeneration of sweat glands impairs body’s temperature regulation and loss of melanocytes can lead to disfigurement. Cellular therapies, including mesenchymal stem cell and growth factor delivery, alongside large-scale bio-fabrication techniques like 3D printing, represent the recent biomedical advances currently revolutionizing skin regeneration and repair processes. The development of sophisticated skin models, standardized protocols to assess skin properties, and the development of living tissue equivalents are some of the major milestones in skin tissue engineering that are serving as an alternative to animal models. Some major ones include the use of engineered human skin constructs, full-thickness skin equivalents, and compromised skin assays [35]. Engineered human skin constructs, including full-thickness skin equivalents, are also used as in vitro systems for drug testing of topically applied cosmetics [36] and to investigate drug delivery and penetration through wounded skin [37].
Tissue engineering encompasses the integration of cells and scaffolds to fabricate functional tissues designed to regenerate and sustain the native architecture and physiological function of damaged tissues and organs. Most studies on skin tissue engineering were primarily focused on the engineering of the epidermis and dermis by culturing keratinocytes and dermal fibroblasts in biodegradable 3D scaffolds. However, primary human epidermal cells being a limited source, efforts have been made to differentiate adipose-derived stem cells, bone marrow-derived mesenchymal stem cells, induced pluripotent stem cells, and other stem cells to epidermal cells [38]. The use of endothelial cells to promote vascularization [39] and melanocytes for pigmentation [40, 41] are among the recent advancements that enhance both the functional and aesthetic outcomes of engineered skin constructs. The dermal and epidermal layers have been engineered separately and coupled to form the bilayer constructs. This often leads to delamination, and approaches to engineer the epidermal-dermal layer simultaneously with a well-integrated epidermal-dermal junction for accelerated wound healing and integration with the host tissue are required.
Scaffolds form an integral part of tissue engineering that provides a structural framework upon which cells can attach, proliferate, and form the 3D microstructure architecture for the tissues. A wide range of natural protein and polysaccharide-based polymers, such as collagen, gelatin, alginate, HA, fibrin, chitosan, and agarose, have gained significant acceptance as hydrogels or scaffolds for skin tissue engineering. These natural biomaterials could mimic the ECM, providing biological cues for enhanced cellular performance and hence can serve as exemplary candidates for scaffold fabrication. The design and selection of an appropriate biomaterial and its physicochemical and biological properties are pivotal in crafting scaffolds for tissue engineering. Ideally, a scaffold for skin tissue engineering should foster favourable cellular interactions and exhibit good mechanical strength and physical properties to support and integrate with the body’s natural processes of wound healing. The characteristic properties expected for 3D scaffolds used for skin tissue engineering are excellent swelling behaviour or fluid absorption property, an interconnected porous network for cell seeding, cell migration, and ECM deposition, appropriate tensile strength, and elastic modulus, the presence of functional groups that can be modified for optimal growth factor and drug release to accelerate re-epithelialization and vascularization. Research is focused on encapsulating strong antioxidants, anti-inflammatory agents, antibiotics, growth factors, and protease inhibitors in 3D scaffolds to accelerate wound healing. Growth factors such as fibroblast growth factor, epidermal growth factor, platelet-derived growth factor, insulin-like growth factor, vascular endothelial growth factor, and transforming growth factor beta are the key signals used to accelerate cell proliferation, migration, ECM remodelling, and angiogenesis. The scaffolds are also a delivery vehicle for antibiotics and antimicrobials to reduce infection during skin wound healing.
Chitosan for wound healing and skin tissue engineering
Chitosan structure & biological properties
Chitosan is a linear natural carbohydrate polymer derived from chitin, the second most abundant natural polymer, through the partial alkaline deacetylation process. Chitin is a linear mucopolysaccharide composed of (1→4) linked 2-acetamido-2-deoxy-β-D-glucopyranosyl units primarily sourced from shells of crustaceans like shrimps, crabs, crawfish etc., whereas chitosan is poly-(β-1/4)-2-amino-2-deoxy-D-glucopyranose biopolymer featuring three different types of reactive functional groups at C–2, C–3 and C–6 position [42] (Figure 2). Molecular weight, porosity, viscosity, degree of deacetylation, and water absorption capacity are crucial among the physicochemical parameters of chitosan. The molecular weight of chitosan can range from 300 kDa to more than 1,000 kDa, depending on the source and method of preparation [43]. Chitosan with high molecular weight and low polydispersity is typically obtained from crustacean sources like shrimp and crab shells, as well as squid bone plates, while chitosan from fungal sources tends to have a lower molecular weight and higher polydispersity [44–46]. In terms of bioactivity, low molecular weight chitosan (< 20 kDa) has more pronounced activity than high molecular weight chitosan (> 120 kDa) [47]. Molecular weight is directly correlated with the degree of deacetylation and inversely correlated with viscosity. The degree of deacetylation refers to the proportion of D-glucosamine units relative to the total amount of N-acetyl-D-glucosamine in the chitosan molecule. Furthermore, this structural parameter impacts its physical and chemical characteristics, which consequently influence the biological performance of the scaffold [48] (Table 2). The viscosity of the chitosan is greatly influenced by the pH of the solvent, degree of deacetylation, ionic strength, molecular weight, concentration of chitosan and temperature of the solvent.
Characteristics of chitosan as a biomaterial
Sl no. | Properties of chitosan as a biomaterial scaffold for engineering skin substitutes and to accelerate wound healing | |
---|---|---|
1 | Biodegradability | Chitosan is amenable to enzymatic hydrolysis by lysozyme & lipase present in the body [49] & bacteria [50] |
2 | Cytocompatibility | Chitosan exhibits good cytocompatibility towards keratinocytes, fibroblasts, dorsal root ganglion neurons, and Schwann cells [51–53] |
3 | Hemostatic effects | Chitosan can adhere to red blood cells and encourages platelets to adhere, activate, and aggregate at the site of vascular injury [54] |
4 | Anti-inflammatory | Chitosan inhibits the release of different pro-inflammatory cytokines such as tumour necrosis factor (TNF)-α & interleukins (IL)-6 [55], and IL-3, 4, 5 [56] |
5 | Angiogenesis | Chitosan can stimulate proliferation of keratinocytes, vascular cells, and dermal fibroblasts [57] |
6 | Mechanical stability | Mechanical properties of CS-based scaffolds can be enhanced through modifications like cross-linking or blending with other natural or synthetic polymers |
7 | Drug delivery | Chitosan has functional groups amenable to coupling bioactive molecules, nanoparticles and drugs for controlled release [58, 59] |
8 | Antibacterial property | The positive charge of chitosan forms electrostatic interactions between the chitosan molecule and the bacterial cell wall |
Chitosan can positively influence various stages of wound healing, including inflammation, proliferation, and remodelling. Chitosan enhances wound healing through a cascade of cellular responses and regenerative pathways. It stimulates fibroblast proliferation and migration, enhances collagen synthesis and deposition to promote tissue granulation, which is crucial for wound closure and tissue repair [60]. Chitosan also stimulates the proliferation and migration of keratinocytes and vascular cells. This process is augmented by chitosan hydrogels, which create a moist environment conducive to cell migration and tissue regeneration. Chitosan can promote the deposition of collagen and other ECM components, which are essential for restoring the structural integrity of the skin. Chitosan also accelerates tissue granulation, a vital step in wound healing involving new blood vessel growth and collagen-rich tissue formation.
Chitosan can modulate both pro- and anti-inflammatory cytokines, helping to regulate the inflammatory response and promote a balanced healing environment. Chitosan modulates cytokine release, stimulating TGF-β, PDGF, IL-1, and IL-8, which drive fibroblast proliferation, collagen synthesis and angiogenesis [61]. However, the effect of chitosan on cytokine production by macrophages is complex, as this is influenced by the concentration and molecular weight of chitosan used and its effect on macrophage polarization. High doses can trigger pro-inflammatory responses, while lower molecular weights may enhance inflammation. High doses of chitosan can activate the NLRP3 inflammasome, leading to increased release of pro-inflammatory cytokines like IL-1β and PGE2 and suppress the release of anti-inflammatory cytokines like IL-1ra and CXCL10/IP-10. Further, it can amplify the secretion of pro-inflammatory cytokines in M1 polarized macrophages. Some studies show that chitosan induces the production of TNF-α, a key pro-inflammatory cytokine. Conversely, chitosan also drives anti-inflammatory responses by influencing macrophage polarization, leading to increased production of anti-inflammatory cytokines like IL-10 and TGF-β1. Other studies have shown that chitosan can inhibit the production of pro-inflammatory cytokines like TNF-α and IL-6. These effects are due to the interaction of chitosan with receptors like CR3, TLR4, and CD14 on macrophages, influencing signalling pathways like NF-κB and JNK, and also modulating the expression of genes such as iNOS, which are involved in inflammation. Chitosan can also help to resolve inflammation by promoting the function of immune cells like polymorphonuclear leukocytes and macrophages, which are essential for clearing debris and bacteria. The cationic nature of chitosan, contributed by the positively charged NH3+ groups of glucosamine, helps it to interact with the negatively charged bacterial cell surfaces, ultimately resulting in impairment of vital bacterial activities, thereby preventing infection.
Chitosan enhances angiogenesis and neovascularization, which is crucial for providing nutrients and oxygen to the wound for healing. Chitosan enhances angiogenesis potentially through mechanisms like stimulating the release of angiogenic factors like VEGF and IL-8. Chitosan can also enhance the proliferation activity and angiogenic capacity of endothelial progenitor cells, which are precursors of endothelial cells and can stimulate the growth of collateral vessels. Chitosan thus enhances wound healing by promoting hemostasis, accelerating inflammation resolution and stimulating cell migration and proliferation, ultimately leading to faster wound closure and tissue regeneration.
Chitosan for wound dressing
Chitosan and its derivatives have been employed in skin and wound care products since 1980 in Japan. Azad et al. (2004) [62] reported chitosan membranes, prepared with a 75% degree of deacetylation and a thickness of 10 µm, in both non-mesh and mesh forms, as a wound dressing in patients. The mesh chitosan membrane demonstrated superior performance compared to the non-mesh form, promoting efficient adherence, hemostasis, re-epithelialization, and reduced itching and pain sensitivity. Histopathological analysis showed that the mesh membrane stimulated skin cell repair and tissue reorganization, whereas non-mesh membranes caused blood accumulation beneath the dressing. Despite the limited sample size, the data strongly support that mesh chitosan membranes are effective in promoting wound healing and tissue regeneration [62].
The mechanical properties of transparent and flexible chitosan membranes were enhanced by incorporating glycerol as a plasticizer. The addition of glycerol significantly improved the tensile strength, swelling rate, water vapor permeability, and wettability of the membranes, while also maintaining long-term stability during enzymatic degradation. To enhance antibacterial properties, tetracycline hydrochloride (TH) and silver sulfadiazine (AgSD) were integrated into the membranes, showing promising controlled-release efficacy and strong inhibition against E. coli and Staphylococcus aureus. Overall, the glycerol-enhanced chitosan membranes, with their sustained drug release and excellent antibacterial activity, have great potential as wound dressings for effective bacterial infection treatment and wound healing [7]. Chitosan was also assessed for its effectiveness as a dressing for split skin graft donor sites, in comparison to conventional dressings. The study showed that chitosan facilitated faster wound re-epithelialization, stimulated capillary and promoted nerve regeneration within the vascular dermis [63].
Chitosan-based composite scaffolds with enhanced physicochemical properties for drug delivery and accelerated cell response
The combination of chitosan with other natural and synthetic polymers to improve physical characteristics and biological properties makes it a promising candidate to accelerate wound healing (Table 3). These combinations can be used to create chitosan-based scaffolds, hydrogels, membranes and electrospun nanofibers, which leverage the benefits of both chitosan and the added polymers, enhancing properties like mechanical strength and biocompatibility. Scaffolds are typically fabricated with a defined porosity and mechanical strength to provide structural support for tissue regeneration. Chitosan-based hydrogels are 3D networks of chitosan chains that can absorb and retain large amounts of water, forming a soft, gel-like material conducive to cell proliferation and migration. Hydrogels often function as injectable materials that fill wound cavities or deliver therapeutic agents. Chitosan hydrogels can be formed through various crosslinking methods. Furthermore, by incorporating specific functional groups or stimuli-responsive elements into the chitosan hydrogel network, researchers can create smart hydrogels that change their properties in response to a particular stimulus, such as pH, temperature, ionic strength, light, etc. This “smart” behaviour allows for controlled release of drugs, targeted delivery and produces dynamic responses according to the environmental changes. Chitosan-based smart hydrogels are discussed separately in the section Smart hydrogels. Chitosan can also be processed to create thin, biocompatible membranes for wound dressings and electrospun nanofibers for enhanced drug delivery and wound healing applications.
Chitosan composites and their research outcomes
Chitosan composite | Purpose | Scaffold type | Cell type used | In vitro cell response | In vivo response | Ref. |
---|---|---|---|---|---|---|
Chitosan/alginate-Hespiridin | Wound healing | Hydrogels | 3T3 murine fibroblast cell line | Cytocompatible, proliferative effect | Alg/Chit hydrogel—incomplete wound healing; Alg/Chit/10% hesperidin—no sign of inflammation and complete wound healing | [64] |
Chitin/chitosan/alginate/fucoidan | Functional wound dressing | Hydrogels | Human dermal fibroblast cells (DFCs) and dermal micro-vascular endothelial cells (DMVECs) | Stimulate cellular proliferation | Granulation and capillary formation on day 7 | [65] |
Chitosan/gelatin hydrogel incorporating PEGMA modified PCL nanofibers/curcumin | Skin regeneration | Hydrogels | L929 mouse fibroblast cells | Biocompatibility, more than 90% cellular viability, higher the PCP, lower the viability | - | [66] |
Chitosan/agarose | Skin substitute in regenerative medicine | Film | BJ human skin fibroblast cells | Non-cytotoxicity and strong cellular adhesion | - | [67] |
Chitosan/fibroin/poly (vinyl pyrrolidone) | Enhanced angiogenesis in wound healing | Hydrogels | - | - | Increased wound healing efficiency with increased fibroin content | [68] |
Polycaprolactone-hyaluronic acid/chitosan-zein | Tissue regeneration | Electrospun nanofiber | NHDF cells | Good cell viability for 7 days, good adhesive & proliferative capacity | - | [69] |
Chitosan-hyaluronic acid/VEGF loaded fibrin nanoparticles | Enhanced angiogenesis in wounds | Sponges | HDF cells and HUVEC cells | More than 85% cell viability | - | [70] |
Chitosan hydrogel/nanocapsules/nanoemulsion loaded with phenytoin | Wound healing | Hydrogel | - | - | Higher percent wound healing for the groups treated with allantoin (C+) and phenytoin-loaded nanocarriers on day 4 | [71] |
Gallic acid/chitosan/hyaluronic (GA-QCS/OHA hydrogels) | Infected wound healing | Hydrogels | L929 mouse fibroblast cells | Better proliferation within 5 days compared to the control group | Accelerated wound healing was obtained due to inhibiting the proinflammatory factor TNF-α and upregulating the vascularization factor CD31 | [72] |
Dual-dynamic-bond cross-linked ferric iron (Fe)/protocatechualdehyde (PA)/quaternized chitosan (QCS) | For closure of skin incisions and promotion of methicillin-resistant Staphylococcus aureus (MRSA)-infected wound healing | Hydrogel | L929 mouse fibroblast cells | Good cytocompatibility | Better incision closure. The activity of QCS and PA, and NIR-assisted ablation, synergistically enhanced the antibacterial capacity of the dressings against MRSA | [73] |
Quaternized chitosan-graft-polyaniline/oxidized dextran | Tissue engineering applications | Hydrogel | ADMSCs and C2C12 myoblast cells | Enhanced proliferation of C2C12 myoblasts | In Sprague-Dawley rats, in vivo hydrogel formation was confirmed | [74] |
Quaternized chitosan-g-polyaniline and benzaldehyde group functionalized poly(ethylene glycol)-co-poly(glycerol sebacate) | Full-thickness skin wound healing | Hydrogels | L929 mouse fibroblast cells | - | Excellent hemostatic performance on the hemorrhaging site | [75] |
Chitosan/xyloglucan composite | Accelerated wound healing | Hydrogels | NIH/3T3 mouse fibroblasts cells | An increase in cell spheroid size and good viability was observed | Displayed continuous degradation in vivo, good wound closure property after 5 days | [76] |
Fluorinated methacrylamide chitosan (MACF) | Diabetic wound healing | Hydrogels | - | - | Good re-epithelialization with MACF + O2 treatment | [77] |
ADMSCs: adipose-derived mesenchymal stem cells; HDF: human dermal fibroblast; HUVECs: human umbilical vein endothelial cells; NHDF: normal human dermal fibroblasts
Chitosan-polymer blend scaffolds
In vitro tests with 3T3 murine fibroblast cells showed enhanced cell proliferation on alginate/chitosan hydrogels loaded with hesperidin, a flavonoid with antioxidant and anti-inflammatory properties. In vivo results revealed that the hydrogels significantly accelerated wound closure compared to the control group treated with gauze [64]. Chitosan-alginate and fucoidan, composite hydrogel sheet (ACF-HS) was designed as a functional wound dressing to create a moist environment for rapid wound healing. This effectively absorbed fluid without maceration, maintaining a constant absorption rate after 18 hours and providing an optimal healing environment. ACF-HS significantly stimulated wound repair, with granulation tissue and capillary formation in rats compared to untreated groups [65].
Curcumin-incorporated composite chitosan-gelatin hydrogels were reinforced with PEGMA-modified polycaprolactone (PCL) nanofibers to enhance hydrophilicity, porosity, mechanical strength, and better integration. The optimal porosity (71.48%–90.43%) and pore size (101–256 μm) were suitable for skin regeneration. L929 skin fibroblasts exhibited good cell attachment and viability in biocompatibility tests. Curcumin in nanofibers further contributed to the scaffold’s regenerative properties [66].
Agarose combined with chitosan functions as an ideal material for creating scaffolds for wound healing and skin regeneration. Chitosan/agarose (CHN/A) films were prepared by neutralization of the mixture to pH 6 and the resulting film was found to be an ideal skin substitute as a cellular graft pre-seeded with patient skin cells [67]. Chitosan and fibroin work synergistically, for optimizing the hydrogel’s swelling properties, angiogenesis, and overall healing efficiency. The hydrogels showed enhanced angiogenesis, particularly with higher fibroin concentrations, as evidenced by the chorioallantoic membrane (CAM) assay. In vivo wound healing studies in mice revealed that hydrogels with higher fibroin content significantly improved wound healing, with a 99.06% reduction in wound size compared to 67.03% in control groups. The hydrogels demonstrated good biocompatibility, with no toxic effects observed in both chick embryos and mice [68].
It is well established that the biological properties of chitosan are closely dependent on its molecular weight and degree of deacetylation. Low molecular weight chitosan-poly(vinyl alcohol) (PVA) soft membranes showed promising antibacterial and wound healing properties. In vitro antibacterial tests demonstrated significant activity against bacterial pathogens, while in vivo wound-healing studies conducted on second-degree burns in rabbits highlighted chitosan’s efficacy as a wound-healing agent. Studies in rabbits revealed normal epidermal growth and accelerated formation of granulation and fibrous connective tissues in the chitosan-treated wounds [6].
A bilayered electrospun membrane, combining the mechanical strength of PCL-HA with the therapeutic benefits of chitosan-zein (CS-ZE) loaded with salicylic acid, was fabricated and evaluated as a potential skin substitute. The PCL-HA upper layer provided mechanical support and acts as a physical barrier, while the CS-ZE bottom layer, loaded with salicylic acid, offers anti-inflammatory and antimicrobial properties (Figure 3A). The bilayered membrane exhibited appropriate porosity, mechanical strength, controlled water loss, and a suitable salicylic acid release profile. In vitro assays using human fibroblast cells showed enhanced adherence, spreading, and proliferation without any cytotoxic effects. Furthermore, no biofilm formation occurred on the membrane’s surface during the experiments [69].
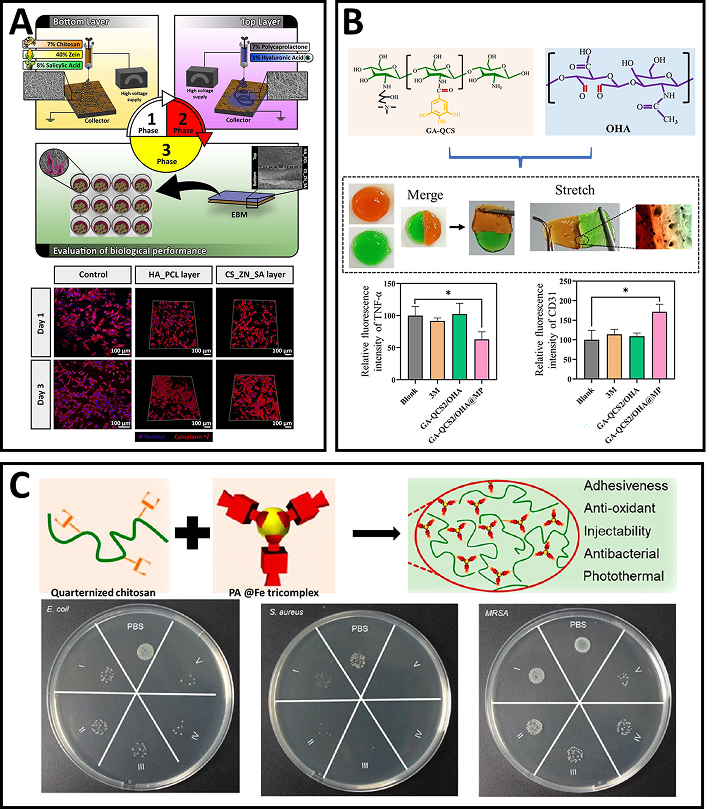
Chitosan-based electrospun scaffolds and self-healing hydrogels for tissue engineering and antimicrobial applications. (A) Schematic representation of the construction of a bilayered electrospun membrane combining chitosan-zein (CS-ZE) loaded with salicylic acid and polycaprolactone-hyaluronic acid (PCL-HA); confocal images of fibroblasts cultured on culture plates and the two layers of the bilayered membrane. Reprinted from [69], Copyright (2016), with permission from Elsevier. (B) GA-QCS2/OHA hydrogels fabricated from GA-QCS copolymer and OHA copolymer; macro and micro self-healing property of the GA-QCS/OHA hydrogel; the GA-QCS2/OHA hydrogel accelerated wound healing by reducing proinflammatory TNF-α levels and upregulating vascularization marker CD31. The components in the subfigure are reprinted from [72], Copyright (2023), with permission from Elsevier. (C) An adhesive hydrogel fabricated using quaternized chitosan and PA@Fe tricomplex; antibacterial properties of the hydrogel against E. coli, Staphylococcus aureus, and MRSA. Adapted from [73], Copyright (2021), with permission from American Chemical Society
Chitosan-based PVA (PVA/CS) dressings were developed to deliver (S)-ibuprofen (IBP) through controlled release using cyclodextrin carriers, aimed at promoting faster skin wound healing. The dressings were fabricated using a supercritical carbon dioxide (scCO2)-assisted technique, which allowed for the sustained release of IBP. In vitro and in vivo studies demonstrated that the IBP-loaded PVA/CS dressings reduced inflammation, prevented scab formation, and accelerated wound healing compared to other treatments. The cyclodextrin carriers ensured a controlled release profile, with the wound size shrinking more rapidly in animals treated with the IBP-loaded dressings, while the anti-inflammatory effect supported skin regeneration. Although further pharmacokinetic studies are needed, these findings suggest that chitosan-based hydrogel dressings with controlled IBP delivery are a promising strategy for accelerating wound healing [78].
Li et al. (2016) [79], probed the mechanism of accelerated wound healing using PVA/COS (chitosan oligosaccharide)-AgNPs nanofibers. COS, a mixture of oligomers composed of β-1,4-linked D-glucosamine residues, was used for the study. COS possesses excellent biocompatibility, biodegradability, hemostatic action, and antibacterial and anti-inflammatory effects. The study reported that the PVA/COS-AgNPs nanofiber accelerated wound healing through the activation of TGFβ1/Smad signalling pathway. In a rat model, treatment with the nanofibers increased the expression of TGFβ1, collagen I, collagen III, and phosphorylated Smad2/3. When the TGFβ1 receptor kinase inhibitor SB431542 was applied, the beneficial effects of the nanofibers on wound healing were inhibited, suggesting the pathway’s essential role in wound healing [79].
Chitosan-based scaffolds were also used for growth factor delivery to enhance angiogenesis during wound healing. Chitosan-HA composite sponges were incorporated with fibrin nanoparticles loaded with VEGF for enhancing wound healing in diabetic patients. VEGF-loaded fibrin nanoparticles (150–180 nm) showed 60% release of VEGF within three days, with a sustained release over seven days, making it suitable for wound healing applications. Cell viability and attachment studies with human dermal fibroblast cells and human umbilical vein endothelial cells (HUVECs) demonstrated that the composite sponge was cytocompatible. HUVECs seeded on VEGF-containing sponges exhibited capillary-like tube formation, a key indicator of angiogenesis. Additionally, the scaffolds showed appropriate mechanical strength, swelling, degradation, and hemostatic potential, all crucial for effective wound dressing materials [70].
The chitosan hydrogels with nano capsules and nano emulsions loaded with phenytoin under appropriate pH and rheological properties, enabled controlled release and improved skin adhesion. In vitro skin permeation studies showed that nanoencapsulation reduced phenytoin’s systemic absorption while maintaining its therapeutic effectiveness in wound healing. Further, phenytoin-loaded nanocarriers enhanced collagen fiber production and fibroblast activity in the wound tissue, supporting its potential as a repurposed drug for skin healing. Nano-encapsulation was found to improve drug retention in the skin without affecting the wound-healing process, minimizing the risks of percutaneous absorption [71].
Chitosan’s abundant amino groups allow for easy modification through cross-linking reactions, making it a versatile material for hydrogel development. Chitosan, when quaternized, exhibits improved biocompatibility, enhanced water solubility, and greater bactericidal activity compared to its unmodified form [80, 81]. In one study, Bai et al. (2023) [72] developed an injectable, self-healing, and multifunctional gallic acid (GA)-quaternized chitosan (QCS)/oxidized hyaluronic acid (OHA) hydrogel with antibacterial, antioxidant, and hemostatic properties for wound healing. The hydrogel, loaded with mupirocin, effectively inhibited the growth of E. coli and S. aureus, and promoted cell migration and tissue regeneration by scavenging reactive oxygen species (ROS). In a rat full-thickness wound model infected with S. aureus, the hydrogel accelerated wound healing by reducing proinflammatory TNF-α levels and upregulating vascularization marker CD31 (Figure 3B). The GA-QCS/OHA hydrogel presents a promising strategy for promoting wound regeneration, especially under complex infection conditions [72]. QCS can also be used to design a dual-dynamic-bond cross-linked adhesive hydrogel with self-healing, antibacterial, and antioxidant properties for efficient wound closure and post-closure care. The hydrogel, incorporating ferric iron (Fe) and protocatechualdehyde (PA), enabled skin incision closure and accelerated healing of methicillin-resistant Staphylococcus aureus (MRSA)-infected wounds (Figure 3C). Its pH-sensitive coordinate bonds and dynamic Schiff base bonds allowed for autonomous healing and on-demand dissolution. The hydrogel demonstrated excellent injectability, biocompatibility, and NIR responsiveness, with strong mechanical strength and tissue adhesiveness. In vivo tests revealed high wound closure effectiveness and significant promotion of healing in MRSA-infected rat wound models [73]. Further modifications of pure QCS led to the development of compounds with enhanced properties, surpassing the performance of the original material. Improved cytocompatibility, increased water solubility and enhanced antibacterial efficacy of QCS-polyaniline copolymer [74] led to the development of self-healing hydrogels based on quaternized chitosan-g-polyaniline (QCSP) and benzaldehyde-functionalized poly(ethylene glycol)-co-poly(glycerol sebacate) (PEGS-FA) were developed as multifunctional bioactive wound dressings with antibacterial, antioxidant, and electroactive properties. These hydrogels exhibited excellent self-healing, electroactivity, free radical scavenging capacity, antibacterial activity, and biocompatibility. The hydrogel with 1.5 wt% PEGS-FA showed optimal in vivo blood clotting and significantly enhanced wound healing in a full-thickness skin defect model, outperforming both QCS/PEGS-FA hydrogels and commercial TegadermTM film. The hydrogels promoted gene expression of growth factors such as VEGF, EGF, and TGF-β, contributing to improved granulation tissue formation, collagen deposition, and overall wound healing. The tunable properties of the hydrogels, including gelation time, stiffness, adhesiveness, and conductivity, made them highly adaptable for wound healing applications. In vivo results showed that the QCSP3/PEGS-FA1.5 hydrogel significantly promoted ECM synthesis and granulation tissue development, leading to enhanced healing. These multifunctional hydrogels hold great promise as effective bioactive wound dressings for full-thickness skin wound healing [75].
Another modified form of chitosan is methacrylated chitosan, where methacrylate groups (derived from methacrylic acid) are chemically attached to the chitosan polymer. Methacrylated chitosan was used to construct a bioactive composite hydrogel designed to promote full-thickness skin wound healing in a mouse model. The hydrogel was synthesized through a Schiff base reaction with aldehyde-containing xyloglucan, forming a dynamic crosslinking network that enabled shear-thinning and self-healing properties for easy injection and in situ gelation. Photo-initiated polymerization of methacrylate groups created a second covalent crosslinking network, providing tunable mechanical properties ideal for clinical application. In both in vitro and in vivo tests, the hydrogel demonstrated significant bioactivity, promoting cell spheroid formation and accelerating wound healing by enhancing re-epithelialization, collagen deposition, angiogenesis, and the formation of hair follicles. When compared to commercially available polyurethane dressings, the hydrogel showed superior wound healing efficiency, including faster wound closure and improved tissue regeneration. The galactoside units in xyloglucan upregulated cell proliferation and supported enhanced tissue formation at the wound site. With its excellent biocompatibility, biodegradability, and cost-effectiveness, this all-polysaccharide hydrogel holds strong potential for future clinical use in wound care. This study marks the first application of a dynamic, bioactive xyloglucan-based hydrogel for wound healing, offering a promising strategy for clinical translation [76].
Fluorinated methacrylamide chitosan (MACF) is another modified form of chitosan, where the chitosan polymer is chemically altered by attaching methacrylamide groups and incorporating fluorine atoms into its structure. A novel wound dressing based on MACF was tested for enhanced oxygenation in a diabetic mouse wound model. The MACF-based hydrogel dressing, MACF + O2, was compared to a commercial hydrogel dressing, AquaDerm, and no treatment controls. MACF + O2 outperformed AquaDerm in promoting wound closure, with histological analysis indicating enhanced collagen synthesis and neovascularization. The MACF + O2 treatment also resulted in improved alignment of collagen fibers, demonstrating better skin tissue maturation compared to AquaDerm and the untreated controls. Nuclear magnetic resonance spectroscopy confirmed that the degradation products of the MACF-based dressing did not accumulate in vital organs such as the lung, liver, or kidney after 14 days, indicating its safety. The study highlights the beneficial role of chitosan in the MACF dressing, enhancing tissue regeneration without causing toxicity. Overall, these findings support the potential of chitosan-based MACF hydrogel dressings as an effective solution for improving wound healing, particularly in diabetic patients [77].
Chitosan-nanoparticle composites
Various metallic nanoparticles (MNPs) and metal oxide nanoparticles are being used for wound-healing applications. Table 4 shows different types of nanoparticles with their chitosan composites. The toxicity of MNPs is a significant concern, which can be effectively addressed by encapsulating them in biocompatible materials such as chitosan. In return, the incorporation of MNPs enhances the matrix by elevating its structural integrity and mechanical performance, including tensile strength, stiffness, and durability. Moreover, chitosan’s antimicrobial properties are amplified in the presence of MNPs [82]. MNPs, particularly those of silver, copper, or zinc, exhibit inherent antimicrobial activity, effectively inhibiting the growth of a broad spectrum of bacteria.
Chitosan/nanoparticle composites and their research findings
Chitosan/nanoparticle composite | Drug/molecule loaded | Purpose | In vitro cell response | In vivo response | Ref. |
---|---|---|---|---|---|
Chitosan/glycosaminoglycan/AgNP electrospun scaffold | - | Antibacterial scaffolds for better wound healing | Good cell viability; antibacterial property against both E. coli and Staphylococcus aureus | - | [83] |
Chitosan-L-glutamic acid (CG) derivative/silver nanoparticles | Hyaluronic acid | Antibacterial wound dressings | Non-toxic to L929 cells size of the inhibition zone increased in a concentration-dependent manner average diameters of the zones for composites against E. coli and S. aureus increased from 20.5–33.0 mm and from 15.5–19.5 mm, respectively | Evidence of healing on day 3, with calluses and slight inflammation | [84] |
Silver nanoparticle-impregnated chitosan-PEG hydrogel | - | Wound healing in diabetes-induced rabbits | The zone of inhibition against E. coli, Pseudomonas aeruginosa, Bacillus subtilis and S. aureus was 20.2 ± 1.0, 21.8 ± 1.5, 15.5 ± 0.8 and 21.5 ± 0.5 mm, respectively | AgNPs incorporated chitosan-PEG hydrogel group was even faster than the healing of wounds with a positive control (32.9 ± 2.1%) | [85] |
Chitosan-loaded silver nanoparticles | Iturin | Antibacterial and wound care applications | Inhibition zone for both E. coli ATCC25922 and S. aureus ATCC29213 | The wound area gradually decreased from the 7th day after the application of sponge dressing | [86] |
PEO/chitosan nanofibers/ZnO | Ciprofloxacin | Burn infection management | Better antibacterial activity of Cip loaded nano webs at 1 μg/mL concentration; ciprofloxacin loaded nano webs 82.5% cell viability HDF cells | - | [87] |
Chitosan/polyethylene glycol/copper film | Naproxen | Anti-infection wound dressing | Cu2+ ions release inhibited biofilm formation; films with 0.1 mM Cu demonstrated better adhesion and proliferation of human skin keratinocytes A341 cell lines | - | [88] |
Copper nanoparticle/chitosan/gelatin composite scaffold | - | Skin tissue engineering application | Scaffolds with copper concentration of 0.01% showed a higher proliferation rate; no oxidative stress upon DCFDA staining. After 7 days, uniform layer of fibroblast cells over the scaffold’s surface | - | [89] |
Chitosan-based copper nanocomposite (CCNC) | - | Wound healing | - | Decreased TNF-α production on 3rd, 7th and 11th day; can decrease the inflammatory reaction; enhanced fibroblast proliferation and collagen deposition; promoted intact re-epithelialization in rats | [90] |
Chitosan/AuNPs composite | - | Antimicrobial wound dressing | Composite was non-toxic to normal human skin cell line BJ-1; maximum inhibition against P. aeruginosa, with inhibition zone diameter of 26 ± 1.8 mm; better antifungal activity against unicellular fungi than multicellular fungi | - | [91] |
Polyacrylic acid/carboxymethyl chitosan/ultrasmall gold nanoparticles (PAA-CMCS-UsAuNPs) | - | Antibacterial hydrogel | Disorder of the surface charge of bacteria and the damage bacterial membrane; ROS production increased in both S. aureus and E. coli to a level of 200% and 300% after hydrogel exposure | On day 4, hydrogel produced significant regeneration of the epidermis in a full-thickness skin wound model. On day 8, a reduction in inflammatory cells | [92] |
Castor oil (CO)/chitosan/ZnO NP (CS/ZnO) | - | Antibacterial wound dressings | Biocidal activity increased with increased CS/ZnO content; stronger bactericidal effect on Gram-positive cells S. aureus and Micrococcus luteus; after 24 h, CS/ZnO loadings ≤ 5.0 wt%, with 90–97% cell; slight increase in cell viability after 72 h of incubation | Wound closure after 14 days in full-thickness wounds on the back of Sprague-Dawley rats | [93] |
Chitosan/CuO-NP | - | Anti-coagulant in wound healing | Cryptococcus neoformans could not grow at 10,000 mg/L concentration of chitosan-NP’s; at 10,000 mg/L, chitosan/CuO nanocomposites inhibited the growth of C. neoformans, B. subtilis, and E. coli; B. subtilis was more sensitive than S. aureus and E. coli; good anticoagulant property | - | [94] |
Chitosan/polyvinyl alcohol/TiO2 composite membranes | - | Wound regeneration | Better hemocompatibility demonstrated by erythrocyte lysis of 3.52%. In vitro wound closure rate of 92.3% at 48 h in fibroblast HIH3T3 cells | - | [95] |
Chitosan/TiO2 composite membrane | - | Wound dressing and skin regeneration | Enhanced L929 proliferation and survival, reduced oxidative stress and apoptosis | - | [96] |
Silver nanoparticles/chitosan composites
Silver is often employed in the form of silver nanoparticles (AgNPs) because of their physicochemical properties, which maintain the bioactive nature of silver and thus exhibit high efficiency as antimicrobial agents in wound healing applications [97]. AgNPs are antibacterial [98] and antifungal [99, 100]. Antioxidant, anti-tumor, anti-angiogenic, and anti-inflammatory activity are also other significant effects of AgNPs [101]. Proliferation of fibroblasts is critical in the healing of chronic skin lesions, especially those prone to infections. Sandri et al. [88] found that AgNP-loaded chitosan/GAG scaffolds could significantly enhance fibroblast proliferation by providing a protection against the cytotoxic effects of AgNPs. These scaffolds also exhibited high antimicrobial activity, particularly against S. aureus [83].
AgNPs can be blended with CS in many forms, like hydrogels, fibers, membranes, films, scaffolds, and sponges, to suit a particular requirement and for added functionality. Both sponge- and film-based chitosan-AgNP composites have been thoroughly investigated for their potential to enhance wound healing [102–104]. Chitosan sponge-like composites containing AgNPs and HA were tested in vivo for wound healing. The composites became transparent hydrogels due to their excellent water-holding capacity, thereby improving their contact with the wound area. This preparation offered continuous antibacterial protection by strongly inhibiting E. coli and S. aureus through AgNP release, with low cytotoxicity to L929 fibroblast cells [84]. Coupled with antibacterial properties, the anti-inflammatory responses also aid in the use of AgNPs in addressing chronic wounds, such as diabetic ones. Chitosan-PEG hydrogel with AgNPs upon in vivo application promoted wound healing in diabetic rabbits due to its slow degradation and sustained AgNP release for seven days or more. Under in vitro conditions, these hydrogels exhibited improved antimicrobial and antioxidant activities when compared to unmodified chitosan-PEG hydrogels. These encouraging findings indicate the potential of the hydrogel in diabetic wound therapy, and subsequent research could involve its mechanism and clinical use in humans [85]. Moreover, agents that could improve the antimicrobial potential could be used along with chitosan/AgNPs to establish better antimicrobial scaffold preparation. Chitosan composite sponge dressing loaded with iturin-AgNPs was developed by Zhou et al. [86] where the scaffolds were characterised with high porosity, good water absorption and antibacterial activity. In vivo results showed that the chitosan dressing loaded with iturin-AgNPs more effectively inhibited bacterial infection and improved wound healing compared to commercial AgNP-loaded dressings. The mechanisms underlying this enhanced healing included accelerated re-epithelialization, collagen formation, and increased antibacterial effects. Importantly, the treatment showed no toxicity to the mice’s organs, making it a promising approach for wound care applications [86].
Despite this potential, the clinical translation of chitosan/AgNPs wound dressings is hampered by the risk of toxic AgNP accumulation at the wound site [105]. Even though the systemic toxicity associated with silver ions is rare, still development of argyria and loss of night vision are some serious complications [106–108]. Also, silver exhibits a dose-dependent toxicity to both cuticle cells and fibroblasts [82]. In certain instances, capping of chitosan could help in overcoming the cytotoxic effects of AgNPs. Studies in rat models have examined the potential of chitosan-capped silver nanoparticles (Ch/AgNPs) to enhance burn wound regeneration and repair by reducing inflammatory response marked by reduced level of IL-1β and neutrophil counts. Additionally, Ch/AgNPs were also capable of promoting rapid re-epithelialization, improving granulation tissue formation, and increasing key growth factors such as TGF-β1 and bFGF. Histopathological, molecular, and biochemical analyses all pointed towards Ch/AgNPs accelerating burn wound healing by shortening the repair phases, suggesting their promise for wound regeneration [109].
Copper nanoparticles/chitosan composites
Besides the stabilization of ECM [87], stimulation of dermal fibroblast proliferation [110], production and secretion of various types of collagens and elastin [111] are some of the major functional roles of copper on the skin architecture. Similar to AgNPs, systemic copper poisoning can also occur at higher doses. At higher doses, these nanoparticles diffuse easily through the bloodstream, triggering potential toxicity [82]. The addition of copper nanoparticles (CuNPs) has a significant effect on the structural aspects of chitosan. CuNP doping to chitosan scaffold prepared through freeze drying improved the scaffold structure extremely suitable for skin tissue engineering [88]. Besides, at higher Cu concentrations, the mechanical strength of the scaffolds increases, which is evident through the study of Kumari et al. [89]. CuNPs are also known for their antibacterial properties. The mechanism is thought to be through the peptidoglycan hydrolysis and also through the competition of Cu with cytoplasmic iron-sulfur enzymes, disrupting their function [112, 113].
Wound healing potential of chitosan-based copper nanocomposite (CCNC) was studied in excision wound model in adult Wistar rats. Pronounced contraction of the wound and increased angiogenesis, fibroblast proliferation, and collagen deposition were observed upon topical application of CCNC. Collagen synthesis and re-epithelialization were also evident through histopathological examination. CCNC treatment resulted in declining TNF-α levels in rats, suggesting that copper helped reduce the inflammatory response induced by chitosan. Immunohistochemistry demonstrated significant enhancement in angiogenesis, substantiating the fact that the copper nanocomposites based on chitosan indeed promoted wound healing by modulating the most critical cell types, cytokines and growth factors [90].
Gold nanoparticle/chitosan composites
Gold nanoparticles (AuNPs) extraordinary properties, such as biocompatibility, facile functionalization, and unique optical features, make them an excellent choice for their applications in various fields such as drug delivery, bioimaging, diagnostics and cancer therapy. Chitosan hydrogels with AuNPs can be used in combating antimicrobial resistance by reducing bacterial infections to promote faster wound healing. Spherical and monodispersed preparations of hydrogel have been tested in vitro, which demonstrated excellent antibacterial and antifungal properties [91]. Besides their exceptional antibacterial properties, controlling the aggregation of AuNPs remains a major challenge that prevents them from being used for wound healing activities. Dong et al. [92] developed a composite material, featuring carboxymethyl cellulose (carboxymethyl chitosan, CMCS), that could effectively limit the self-aggregation of AuNPs to sizes of 1–3 nm. This composite could also attract bacteria with negatively charged surfaces, resulting in enhanced antibacterial efficacy [92].
Metal oxide nanoparticles
Metal oxides such as zinc oxide (ZnO), titanium oxide (TiO2) and copper oxide (CuO) are some of the heavily researched materials for wound healing applications, where ZnO and TiO2 has wound healing potential [114] and CuO serving as good antibacterial agents which further enhances the healing process [115].
Zinc oxide nanoparticles (ZnO NPs)/chitosan composites: ZnO NPs are also known for their antibacterial property, which occurs primarily through the release of Zn ions and also through the photocatalytic generation of hydrogen peroxide. The combination of chitosan and ZnO demonstrated enhanced antimicrobial efficacy, exhibiting an inhibition zone 5–15 mm larger than that of chitosan alone, while ZnO treatment caused cell membrane rupture in MRSA and cell shrinkage in Pseudomonas aeruginosa [116]. The antibacterial activity of chitosan-based nanofibers has been enhanced by incorporating low concentrations of ZnO NPs and ciprofloxacin. Optimization via response surface methodology yielded nanofibers with a diameter of 116 nm and a small standard deviation of 21 nm. These ZnO-loaded nanofibers exhibited improved thermal stability and a pH-dependent drug release profile, with faster release in acidic environments and slower release at physiological pH (7.4). The release kinetics followed a zero-order model at pH 7.4 and the Hixson Crowell model at pH 5.5. Antibacterial activity was enhanced, and the nanofibers exhibited non-toxicity to human dermal fibroblast and keratinocyte cell lines, with cell viability rates of 82.5% and 85.6%, respectively. The addition of ZnO NPs and ciprofloxacin increased the nanofiber diameter and antibacterial efficiency, with a zone of inhibition ranging from 15 to 25 mm. These nanofibers, with good thermal stability, sustained drug release, and enhanced antibacterial properties, show promise as an effective solution for preventing or treating burn wounds [117]. Furthermore, the optimized healing performance of full-thickness wounds on Sprague-Dawley rats is determined solely based on the synergistic effects of its composite components, particularly the ZnO concentration. The epithelialization period was reduced to 15.5 days for castor oil (CO)/5% wt chitosan-ZnO scaffold compared to 16.2 days in the case of CO-treated wounds and 20.3 days in the gauze-treated control [93].
Copper oxide nanoparticles (CuO NPs)/chitosan composite: Ahmed et al. (2021) [94] used chitosan/CuO nanocomposite as an anticoagulant to address the first step of wound healing, the hemostasis. Although limited data exists on the interaction between CuO NPs and chitosan in skin fibroblasts, there is considerable information available regarding the effects of copper oxide on the skin. Histological analysis showed normal skin structure with intact epidermal and dermal layers, but copper-treated explants, particularly those exposed to 1 µmol/L copper ions, exhibited high collagen and elastin fibre deposition, especially in the mid-dermal layer. Furthermore, the levels of TGF-β1, a key growth factor, increased significantly (~2.5- to 4-fold) in the culture medium after 4 and 6 days of exposure to copper ions. copper treatment led to a dramatic increase in elastin secretion, with levels almost doubling those of controls after just 1 day, and remaining significantly elevated after 4 and 6 days. Pro-collagen I levels also increased by approximately 20% after 1 day of exposure to copper ions, with higher levels observed at 0.02 µmol/L after 6 days of incubation. No significant changes in HA levels, a GAG, were detected after 4 and 6 days of copper treatment [118].
Titanium dioxide nanoparticles (TiO2 NPs)/chitosan composite: TiO2 NPs facilitate wound healing through their antimicrobial property, where Ti2+ ions work [119] along with ROS to cause changes in the bacterial cell membrane, affecting bacterial DNA replication [120]. Chi/PVA/TiO2 film antimicrobial effect upon SEM imaging confirmed that the morphologies of Bacillus subtilis (Gram-positive bacteria) and E. coli (Gram-negative bacteria) exhibited a great reduction in microbial size with fractured surfaces. Such films were also shown to exhibit good hemocompatibility, biocompatibility and no toxic effect with enhanced cellular migration for wound healing purposes [95]. Membranes based on chitosan/titanium dioxide (CS/TiO2) composite revealed a strong O–Ti–O bonding between TiO2 and chitosan, enhancing the membranes’ porosity, mechanical strength, and flexibility. Improved cellular proliferation, reduced oxidative stress, and decreased apoptosis in L929 fibroblast cells highlighted the in vitro wound healing potential of the membrane. Furthermore, its functional integrity, along with good antimicrobial properties against S. aureus, suggests the potential as an effective wound dressing material [96].
Smart hydrogels
Smart chitosan-based hydrogels are a cutting-edge material that combines the natural biopolymer chitosan with the versatile properties of hydrogels to create a responsive, multifunctional system. These hydrogels are “smart” because they respond to external stimuli such as pH, temperature, ionic strength, or the presence of specific enzymes, enabling them to change their physical or chemical properties. Based on their responsiveness to external stimuli, smart chitosan hydrogels are categorized into pH-sensitive hydrogels, thermosensitive hydrogels, and photosensitive hydrogels [121]. Furthermore, magnetic field corresponding hydrogel, electric field responsive hydrogel and plasma-activated hydrogel are also present, but more scientific validation is required to fully establish their potential, both in vitro and in vivo.
Thermo-responsive chitosan hydrogels
Thermal-responsive hydrogels are polymeric networks that undergo substantial and reversible changes in their structure and physical state in direct response to fluctuations in temperature. These hydrogels are highly sensitive to even minor temperature variations, allowing them to adapt by dynamically altering their morphology or phase as the temperature fluctuates within the surrounding environment [121]. With the pioneering work of Chenite et al. [122] chitosan-based thermogelling systems were introduced in 2000, wherein they developed an innovative injectable, neutral, and thermally responsive gel-forming aqueous solution comprised of chitosan and glycerophosphate (CS/GP) [122].
Chitosan, in its native form, lacks inherent thermosensitivity and does not exhibit temperature-responsive behaviour without incorporating specific additives [122]. Agents like β-GP, hydroxypropyl methylcellulose (HPMC) and poloxamer (PF-127) facilitate the thermosensitive modification of chitosan [123]. GP is an endogenous component in the body that exhibits no cytotoxicity [124]. A chitosan solution acquires thermoresponsive properties at physiological pH upon adding GP, triggering a marked alteration in its behaviour [122]. During the cytotoxic evaluation of the hydrogels, it was found that the CS/GP formulations demonstrated cytotoxicity in a concentration-dependent manner, with increased GP levels, making the CS/GP hydrogel more toxic to cells [125]. However, such preparations exhibit slower sol/gel transitions, leading to delayed gelation upon injection. One approach to improve the properties of so-developed hydrogel is through the addition of agents such as TEMPO-oxidized cellulose nanofiber (TOCNF) that could potentially minimize the steric hindrance. An injectable thermosensitive CS/GP hydrogel integrated with various concentrations of TOCNF was developed and evaluated for in vitro and in vivo responses. TOCNF improved the gelation properties and biocompatibility of the hydrogel, with higher TOCNF concentrations leading to faster sol/gel transitions, a more porous surface, and quicker degradation. MC3T3-E1 and L929 cells exhibited better survival, growth, and attachment on chitosan/TOCNF (CS/TOCNF) hydrogels compared to chitosan alone, with 0.4% TOCNF enhancing cell infiltration in vivo. Despite an initial inflammatory response in Sprague-Dawley rats, the presence of alternatively activated macrophages after two weeks indicated the promising biomedical potential of CS/TOCNF hydrogels, particularly at 0.4% TOCNF [125].
In another innovative approach, the thermosensitive hydrogel was formulated using HPMC, and glycerol with chitosan. HPMC is a propylene glycol ether of methylcellulose, known for its characteristic property of thermoreversible gels. In HPMC, the hydrophilic groups form hydrogen bonds with water at low temperatures, resulting in the formation of cage-like structures that encapsulate the water molecules. HPMC significantly contributes to strengthening intermolecular interactions owing to its elevated polysaccharide content. The inclusion of glycerol reduces the water sheaths surrounding the polysaccharide polymers, facilitating the formation of hydrophobic domains that drive gelation upon heating, ultimately resulting in the establishment of a robust network structure. The hydrogel, distinguished by its low cytotoxicity, biodegradability, and pH 7.4-controlled release, showcases the significant potential for diverse biomedical applications, with HPMC playing a pivotal role in its thermosensitive behaviour and gel formation [126].
Standard injectable hydrogels struggle to adhere to bleeding sites within the body’s moist environment, hindering wound healing and blood clotting. To address this, catechol-hydroxybutyl chitosan (HBCS-C) was developed that exhibited enhanced properties, including temperature-sensitive gelation, injectability, strong tissue adhesion, biodegradability, and biocompatibility. This gel had an excellent liquid-gel transition at different temperatures, through the changes of hydrophilic-hydrophobic interaction and hydrogen bonds generated from hydroxy butyl groups. Upon injection, HBCS-C forms a stable gel directly at the bleeding site, effectively stopping haemorrhage through strong interactions between its catechol and amino groups and the surrounding tissue [127] (Figure 4A).
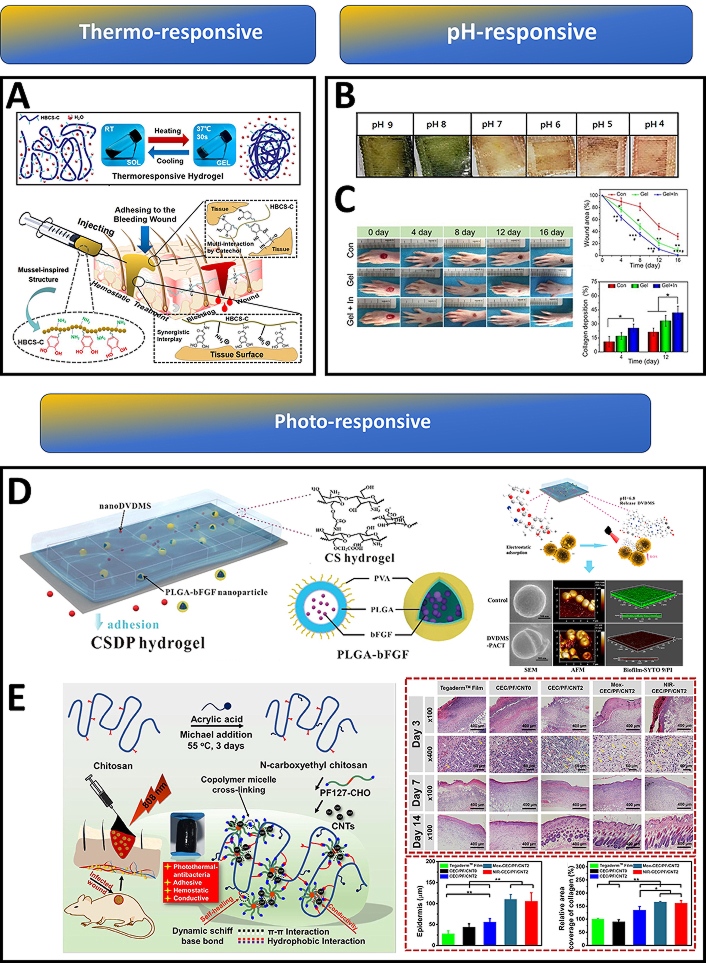
Chitosan-based smart hydrogels for advanced wound management. (A) Schematic illustration of HBCS-C (catechol-hydroxybutyl chitosan) thermoresponsive adhesive hydrogels. Upon injection into the bleeding wound, the thermosensitive HBCS-C hydrogel precursor solution underwent in situ gelation within 30 seconds; tissue adhesive behaviour of HBCS-C hydrogels. Reprinted from [127], Copyright (2020), with permission from American Chemical Society. (B) Smart therapeutic pH-sensitive wound dressing based on red cabbage extract and chitosan hydrogel at different pH values (4–9). Reprinted from [128], Copyright (2021), with permission from Elsevier. (C) Images of the wound area injected with a self-healing and pH-responsive N-chitosan/HA-ALD hydrogel; wound closure was faster and collagen deposition was improved in the insulin-loaded hydrogel group when compared to the control group. Reprinted from [129], Copyright (2021), with permission from Elsevier. (D) Schematic illustration of multifunctional CSDP hydrogel; CSDP hydrogel promoted the release of a dimeric photosensitizer (DVDMS) to a lower pH value in wound areas, thus enhancing its accumulation in bacteria. SEM, AFM, and confocal images showed that these photosensitizers could destroy bacterial membranes and biofilms. Reprinted from [130], Copyright (2020), with permission from American Chemical Society. (E) Schematic representation of the CEC/PF/CNT hydrogel preparation; images of the histomorphological evaluation of the regenerated tissue after healing for 3 days, 7 days and 14 days, compared with the commercial Tegaderm film, the hydrogel groups increased thicknesses of the newly formed epidermis; elevated collagen deposition was observed in the hydrogel groups. Reprinted from [131], Copyright (2020), with permission from Elsevier
pH-responsive chitosan hydrogels
Whenever skin damage occurs, the normal acidic pH of healthy skin shifts to alkalinity (7.4–9) under the influence of reproducing bacterial colonies. In such situations, the monitoring of wound healing status with respect to changing pH is necessary since it influences a number of steps ranging from the protease activity to the angiogenetic potential at the damaged area [132–136]. Dyes that can change colour under pH variation are active components of most pH-responsive hydrogels. Phenol red is one such agent, and when combined with carbon quantum dots (CQDs), was effectively used for the manufacture of polyacrylamide-quaternary ammonium/chitosan hydrogels to assess pH real time. Such hydrogels could indicate the variability in pH in both UV and visible backgrounds [137]. Arafa et al. [128] developed a therapeutic pH-sensitive wound dressing hydrogel using chitosan and encapsulating red cabbage extract (RCE). As any change in pH occurs (from 9 to 4), RCE undergoes colour changes from green to red, respectively, indicating the healing process [128] (Figure 4B).
The development of smart hydrogels that can carry out the controlled release of drugs in response to pH change is another active part of chitosan research. Chitosan-coumarin (CA) hydrogels are used for the controlled release of taxifolin (TFL), the cumulative release of TFL was 80% at low pH (pH 4) and was reduced to 50% at higher pH (pH 9.2). This hydrogel material also retains 99.9% of its self-healing ability in addition to its structural and functional integrity [138]. Not only chitosan, but also its derivatives such as QCS modified with glycidyltrimethyl-ammonium chloride (GTMAC) along with oxidized dextran (OD) and polydopamine-coated polypyrrole nanowires (PPY@PDA NWs) could also act as carriers of antibacterial agents like tobramycin (TOB). pH responsiveness and slow release of TOB is made possible through the Schiff base cross-linking between the OD and TOB entities. The presence of QCS/OD/TOB/PPY@PDA9 hydrogels in models for P. aeruginosa-infected burn wounds could result in enhanced collagen deposition and vascularization with better wound closure [139].
Insulin hormone-based therapy plays a crucial role in addressing diabetic foot ulcers (DFUs), a characteristic chronic wound. Due to the short life span and reduced biological activity of insulin in a peptidase-rich wound environment, topical administration of insulin does not produce a desirable outcome [140]. To overcome this limitation, the insulin was successfully incorporated into N-carboxyethyl chitosan (N-chitosan) based self-healing, injectable hydrogel, allowing its sustained and pH-responsive releasing for up to 14 days. This hydrogel reduced the inflammatory response and enhanced granulation tissue formation (Figure 4C). Augmented collagen deposition, and re-epithelialization were also achieved thereby promoting DFUs healing [129].
Photo-responsive chitosan hydrogels
The application of UV light to a photo-crosslinkable chitosan (Az-CH-LA) aqueous solution rapidly formed an insoluble, flexible hydrogel within 60 seconds. This hydrogel was highly effective in stopping bleeding from a cut mouse tail within 30 seconds of UV irradiation and could strongly adhere two pieces of skin together. When applied to full-thickness skin incisions on mice, the chitosan hydrogel significantly accelerated wound contraction and closure. Histological analysis revealed advanced granulation tissue formation and epithelialization in the hydrogel-treated wounds. The chitosan hydrogel demonstrated excellent wound occlusion and tissue adhesive properties, making it a promising dressing for urgent haemostasis situations. Additionally, its binding strength to skin slices was superior to fibrin glue, highlighting its potential as a tissue adhesive. Overall, the chitosan hydrogel accelerated wound healing in a mouse model, suggesting its potential for use in both wound healing and urgent haemostatic applications [141].
Photodynamic therapy (PDT) finds application in the treatment of wounds with bacterial infection, where PDT helps in faster wound healing through bacterial destruction. Low ROS production in PDT can facilitate the repair and regeneration of damaged tissue through cell proliferation and differentiation [142]. In light of this scenario, photo-responsive hydrogels are being used to combat wound infections involving resistant bacterial species. MRSA considered a priority pathogen by WHO, poses an acute threat to humans. A multifunctional CHDTA hydrogel was developed through the crosslinking reaction between CMCS and HA. Incorporation of polydopamine (PDA) caused the hydrogel to gain its photothermal therapy (PTT) potential at 660 nm and 808 nm laser irradiation. Moreover, this CHDTA is also capable of producing PDT and NO-releasing capability upon adding photosensitizer (TAPP) and nitric oxide donor (L-Arg) onto the network. Laser irradiation at 660 nm, production of singlet oxygen, which subsequently caused L-Arg to release NO [143]. Similarly, CSDP hydrogel with CMCS and sodium alginate (SA) encapsulating DVDMS and poly(lactic-co-glycolic acid) (PLGA)-bFGF displayed remarkable antibacterial activity (99.99% removal) against MDR-S. aureus under mild photoirradiation (30 J/cm2, 5 min) [130]. The repeated PDT along with bFGF could significantly decrease bacterial colonization and help in improving the wound healing process (Figure 4D).
Further, N-carboxyethyl chitosan (CEC) and benzaldehyde-terminated Pluronic F127/carbon nanotubes (PF127/CNT) were used to develop a self-healing and adhesive nanocomposite hydrogel with a remarkable photothermal antibacterial property specifically for infected wounds (Figure 4E). An in vivo experiment in a mouse full-thickness skin wound-infected model indicated that the hydrogels had an excellent treatment effect, leading to significantly enhanced wound closure healing, collagen deposition, and angiogenesis [131].
Table 5 outlines the biological activity of chitosan-based multiresponsive smart hydrogels, highlighting their dynamic response to physiological cues and therapeutic delivery capabilities. Their inherent biological activity stems from carefully engineered functionalities that promote tissue repair and regeneration through a cascade of controlled and synergistic mechanisms. By tailoring the material composition and design, these hydrogels can be customized to achieve specific therapeutic goals, paving the way for advanced regenerative therapies.
Properties and applications of chitosan-based smart hydrogels
Types of CS hydrogels | Examples | Biological activity | Applications | Ref. |
---|---|---|---|---|
Thermo-responsive | CS/TOCNF | Good cytocompatibility and anti-inflammatory biomaterial | Wound healing | [125] |
CS/hydroxypropyl methylcellulose/glycerol | Good fluidity, biodegradability, low cytotoxicity, controlled release | Drug delivery | [126] | |
HBCS/C | Thermosensitivity, injectability, tissue-adhesion, biodegradation, biocompatibility, anti-haemorrhaging barriers | Wound hemostasis | [127] | |
pH-responsive | Polyacrylamide/quaternary ammonium CS/CQDs/phenol red hydrogels | Hemostatic, adhesive properties, wound moisture maintenance, wound healing via antibacterial activity, skin repair function | Wound healing and skin repair function, real-time evaluation of the wound dynamics | [137] |
Methacrylated CS/red cabbage extract hydrogel | Good swelling property, better in vitro release rates, suitable for monitoring visual changes in the wound bed | Wound dressing application | [128] | |
CS/CA/TFL | Biodegradability, cytocompatibility, structural and functional integrity, controlled and local drug-release ability, tunable for the shape of the hydrogel | Drug delivery | [138] | |
QCS/OD/TOB/PPY@PDA NWs | Slow drug release, NIR irradiation-assisted bactericidal activity, inflammation regulation, collagen deposition, vascular generation, and earlier wound closure | Bacterial-infected burn wound healing | [139] | |
N-carboxyethyl CS/HA-ALD/ADH | Compatibility, porous structure, anti-inflammatory, granulation and tissue formation, collagen deposition, accelerated re-epithelialization, neovascularization, improved peripheral neuropathy | Wound dressing in DFUs for healing | [129] | |
Photo-responsive | CMCS/HA/PDA/TAPP/L-Arg | Injectable, adhesive, cytocompatibility, antibacterial properties | Wound healing in MRSA-infected animal wound models | [143] |
CMSC/sodium alginate/DVDMS/PLGA/bFGF nanospheres | Fluorescence imaging, antibacterial activity, inhibiting biofilm formation, biocompatible, promoting epithelialization | Burn infections | [130] | |
CEC/benzaldehyde-terminated PF127/CNT | Good gelation time, stable mechanical properties and hemostatic properties, high water absorbency, and good biodegradability | Infected full-thickness skin wounds | [131] |
ADH: adipic acid dihydrazide; bFGF: basic fibroblast growth factor; CA: coumarin; CEC: N-carboxyethyl chitosan; CS: chitosan; CMCS: carboxymethyl chitosan; CNT: carbon nanotubes; CQDs: carbon quantum dots; DFUs: diabetic foot ulcers; DVDMS: porphyrin photosensitizer sinoporphyrin sodium; HA: hyaluronic acid; HA-ALD: hyaluronic acid-aldehyde; HBCS-C: catechol-hydroxybutyl chitosan; L-Arg: L-Arginine; NWs: nanowires; OD: oxidized dextran; PDA: polydopamine; PLGA: poly(lactic-co-glycolic acid); PPY: polypyrrole; QCS: quaternized chitosan; TAPP: 5,10,15,20-tetrakis (4-aminophenyl) porphyrin; TFL: taxifolin; TOB: tobramycin; TOCNF: TEMPO-oxidized cellulose nanofiber
Clinical translation of chitosan-based scaffolds
The clinical translation of chitosan-based scaffolds, however, is subject to rigorous evaluation through clinical trials. These trials are essential to establish the safety and efficacy of chitosan-based products in humans. This is due to reported instances of allergic reactions related with products containing chitosan. The allergic reactions are mainly due to protein contaminants rather than the chitosan polymer itself. Currently, while numerous preclinical studies showcase chitosan’s potential, the number of commercially available chitosan-based scaffolds is relatively limited. Chitosan’s hemostatic properties are utilized in several commercial products, including HemCon Dental Dressing, ChitoSAMTM 100 and ChitoFlex®, CeloxTM (OMNI-STATTM) and Axiostat®, all proven effective in reducing bleeding time, even in arterial hemorrhages. For burn victims with severe skin loss, chitosan biomaterials offer critical support against fluid loss and infection. Various clinical studies conducted for the treatment of chronic wounds demonstrate that chitosan-based dressings provide uniform wound adherence, essential for successful healing. These dressings also promote hemostasis, rapid re-epithelialization and the formation of healthy, aesthetically acceptable tissue [144]. Concerning wound healing and skin regeneration, clinical trials have explored chitosan’s use in various forms, including wound dressings and gels for the treatment of chronic wounds, surgical incisions and burns; chitosan scaffolds for the regeneration of skin and chitosan-based drug delivery systems for localized wound healing and tissue regeneration. Until now, over 100 clinical studies have been underway, including trials on: SurgiShield gel—an adhesion inhibitor formulated with chitosan—in promoting wound healing following endoscopic sinus surgery, a collagen and chitosan porous sponge scaffolds as a skin substitute for the treatment of difficult-to-heal wounds and EGF-loaded chitosan dressings to facilitate healing and prevent scar formation of cesarean wounds [145]. Despite numerous patents and research, chitosan product translation is slow, due to challenges in understanding the biological interactions of chitosan polymers, scaling up and production and establishing quality control. Rigorous assays, toxicology studies and clear guidelines are crucial for successful clinical translation.
Conclusion and future prospects
In conclusion, this review has explored the significant potential of chitosan-based composite scaffolds for accelerating epidermal-dermal wound healing. Chitosan, with its inherent biocompatibility, biodegradability, and hemostatic properties, holds significant promise for developing scaffolds for engineering epidermal and dermal skin substitutes and wound healing applications. However, bare chitosan scaffolds often suffer from limitations such as poor mechanical strength, rapid degradation, and limited control over drug release. To overcome these drawbacks, researchers have focused on engineering composite scaffolds by combining chitosan with other polymers (both natural and synthetic), nanoparticles, and smart hydrogels. These modifications have demonstrably enhanced scaffold properties, leading to improved adsorption of wound exudates, controlled drug delivery, enhanced antibacterial activity, modulated cell response (including proliferation, migration and differentiation) and tailored anti-inflammatory properties. The incorporation of specific bioactive agents and growth factors further contributes to accelerated tissue regeneration and faster wound closure. By carefully selecting and combining different components, researchers can fine-tune the scaffold’s characteristics to match the specific requirements of the wound healing process.
Looking ahead, the field of chitosan-based wound healing scaffolds is poised for further advancement in the application of 3D bioprinting of skin. This technique enables the precise fabrication of scaffolds with complex architectures that mimic the natural skin structure. Chitosan-based bioinks, often blended with other polymers or modified with nanoparticles, can be precisely deposited to create scaffolds with tailored mechanical properties and degradation rates. Furthermore, bioprinting of multiple cell types and the precise control over scaffold architecture allows for the creation of gradients in mechanical properties and biochemical cues, mimicking the natural skin microenvironment and guiding cell behavior. The incorporation of growth factors and other bioactive molecules into the bioink allows for localized and controlled delivery, promoting angiogenesis and accelerating wound healing. Researchers have explored various bioink formulations, including chitosan blended with collagen, gelatin, HA or other polymers, to optimize printability and scaffold properties. The incorporation of skin cells, such as fibroblasts and keratinocytes, into these scaffolds has shown promising results in promoting skin regeneration in vitro and in vivo [146, 147]. These bioprinted constructs have demonstrated improved wound closure, reduced scar formation and enhanced re-epithelialization compared to traditional wound dressings.
Despite the significant progress made in this field, several challenges remain. Optimizing bioink formulations for printability and biocompatibility is crucial. The mechanical properties of the scaffold must be carefully tuned to match the surrounding tissue and withstand physiological stresses. Ensuring adequate vascularization of the thick bioprinted constructs is essential for nutrient delivery and waste removal. Furthermore, translating these promising results from preclinical studies to clinical applications requires rigorous testing and validation. 3D bioprinting of cell-incorporated chitosan-based scaffolds holds immense potential for revolutionizing skin tissue engineering and wound healing. While challenges remain in optimizing the printability of chitosan-based bioinks, researchers are exploring strategies such as modulating chitosan viscosity through blending with other polymers or incorporating additives to achieve the necessary flowable characteristics for printing and post-printing photo-crosslinking of the engineered construct. We believe that continued research and development in this area will pave the way for the next generation of advanced wound-healing therapies, ultimately leading to improved patient outcomes.
Abbreviations
ACF-HS: | chitosan-alginate and fucoidan, composite hydrogel sheet |
AgNPs: | silver nanoparticles |
AuNPs: | gold nanoparticle |
CCNC: | chitosan-based copper nanocomposite |
Ch/AgNPs: | chitosan-capped silver nanoparticles |
CMCS: | carboxymethyl chitosan |
COS: | chitosan oligosaccharide |
CS/GP: | chitosan and glycerophosphate |
CS/TOCNF: | chitosan/TEMPO-oxidized cellulose nanofiber |
CS-ZE: | chitosan-zein |
CuNPs: | copper nanoparticles |
CuO NPs: | copper oxide nanoparticles |
DFUs: | diabetic foot ulcers |
ECM: | extracellular matrix |
GAGs: | glycosaminoglycans |
GA-QCS/OHA: | gallic acid-quaternized chitosan/oxidized hyaluronic acid |
HA: | hyaluronic acid |
HBCS-C: | catechol-hydroxybutyl chitosan |
HPMC: | hydroxypropyl methylcellulose |
HUVECs: | human umbilical vein endothelial cells |
IBP: | ibuprofen |
MACF: | fluorinated methacrylamide chitosan |
MNPs: | metallic nanoparticles |
MRSA: | methicillin-resistant Staphylococcus aureus |
OD: | oxidized dextran |
PCL: | polycaprolactone |
PDT: | photodynamic therapy |
PEGS-FA: | benzaldehyde-functionalized poly(ethylene glycol)-co-poly(glycerol sebacate) |
PPY@PDA NWs: | polydopamine-coated polypyrrole nanowires |
PVA/CS: | chitosan-based poly(vinyl alcohol) |
PVA: | poly(vinyl alcohol) |
QCS: | quaternized chitosan |
QCSP: | quaternized chitosan-g-polyaniline |
RCE: | red cabbage extract |
ROS: | reactive oxygen species |
TFL: | taxifolin |
TiO2 NPs: | titanium dioxide nanoparticles |
TOB: | tobramycin |
TOCNF: | TEMPO-oxidized cellulose nanofiber |
ZnO NPs: | zinc oxide nanoparticles |
Declarations
Author contributions
ASD: Writing—original draft, Writing—review & editing. NM and RM: Conceptualization, Writing—review & editing. All authors read and approved the submitted version
Conflicts of interest
The authors declare that they have no conflicts of interest.
Ethical approval
Not applicable.
Consent to participate
Not applicable.
Consent to publication
Not applicable.
Availability of data and materials
Not applicable.
Funding
ASD acknowledges Kerala State Council for Science, Technology and Environment (KSCSTE), Thiruvananthapuram, Kerala, India, for providing financial support through the fellowship. The funders had no role in study design, data collection and analysis, decision to publish, or preparation of the manuscript.
Copyright
© The Author(s) 2025.
Publisher’s note
Open Exploration maintains a neutral stance on jurisdictional claims in published institutional affiliations and maps. All opinions expressed in this article are the personal views of the author(s) and do not represent the stance of the editorial team or the publisher.