Abstract
Despite recent advancements in the field of neuro-ophthalmology, the rising rates of neurological and ophthalmological conditions, mismatches between supply and demand of clinicians, and an aging population underscore the urgent need to explore new therapeutic approaches within the field. Glucagon-like peptide 1 receptor agonists (GLP-1RAs), traditionally used in the treatment of type 2 diabetes, are becoming increasingly appreciated for their diverse applications. Recently, GLP-1RAs have been approved for the treatment of obesity and recognized for their cardioprotective effects. Emerging evidence indicates some GLP-1RAs can cross the blood-brain barrier and may have neuroprotective effects. Therefore, this article aims to review the literature on the neurologic and neuro-ophthalmic role of glucagon-like peptide 1 (GLP-1). This article describes GLP-1 peptide characteristics and the mechanisms mediating its known role in increasing insulin, decreasing glucagon, delaying gastric emptying, and promoting satiety. This article identifies the sources and targets of GLP-1 in the brain and review the mechanisms which mediate its neuroprotective effects, as well as implications for Alzheimer’s disease (AD) and Parkinson’s disease (PD). Furthermore, the preclinical works which unravel the effects of GLP-1 in ocular dynamics and the preclinical literature regarding GLP-1RA use in the management of several neuro-ophthalmic conditions, including diabetic retinopathy (DR), glaucoma, and idiopathic intracranial hypertension (IIH) are discussed.
Keywords
Glucagon-like peptide 1, incretins, neuro-ophthalmology, neuroprotectionIntroduction
Neuro-ophthalmology encompasses the spectrum of neurologic, ophthalmic, and systemic disorders affecting the optic nerve, central visual pathways, or neural control of eye movements. With an interdisciplinary focus, practitioners utilize ophthalmic imaging, neuroimaging, laboratory evaluation, electrophysiology, pathologic evaluation, and genetic testing to evaluate patients. With an aging population and an increase in demand for both neurologic and ophthalmic services, it is expected that the demand for neuro-ophthalmologists will continue to outstrip supply [1–3]. A recent survey from the North American Neuro-Ophthalmology Society (NANOS) recently demonstrated that the median wait time to receive care for new patients, many of whom present with acute concern, is 6 weeks [1]. These trends are similar worldwide, as shown by a recent study demonstrating only two of fifteen countries surveyed had adequate access to neuro-ophthalmology [4]. Such projections demonstrate the critical need to reimagine care and therapy within the field.
Despite these shortages, the practice of neuro-ophthalmology has changed rapidly over the course of the last decade. Breakthroughs in disease diagnosis (i.e. identification of myelin oligodendrocyte glycoprotein 10 antibodies in optic neuritis subclasses) [5], advances in imaging (i.e. utilizing macular ganglion cell inner plexiform layer thickness to predict visual outcomes in chiasmal compression) [6], enhancements in surgical management [i.e. venous sinus stenting for idiopathic intracranial hypertension (IIH)] [7], and novel therapeutics (i.e. viral vector gene therapy for Leber’s hereditary optic neuropathy) [8] are forging the path ahead. As the mechanisms underlying disease pathology are unveiled, various therapeutics are being repurposed for their use in neuro-ophthalmology. Recently, there has been mixed success in the use of rituximab for the treatment of Grave’s ophthalmology [9, 10]. With the goal of enhancing patient outcomes and enabling practitioners to overcome physician supply shortages, there is an urgent need to explore new pharmacologic therapies that target mechanisms of neuro-ophthalmic degeneration.
One drug class that has garnered interest in the management of neuro-ophthalmic conditions is glucagon-like peptide 1 receptor agonists (GLP-1RAs). Principally used in the management of type 2 diabetes mellitus (T2DM) and obesity, recent work posits a broader role of the peptide in modulating numerous physiologic pathways [11, 12]. In this review, the application of GLP-1RAs in neuro-ophthalmology is discussed in detail. Moreover, the role of endogenous glucagon-like peptide 1 (GLP-1) in the body is reviewed, including neuronally synthesized GLP-1, with a focus on how recent research developments may point towards therapeutic utility of GLP-1RAs in neuro-ophthalmology.
GLP-1 overview
Incretins, which increase insulin production and reduce blood glucose levels, are hormones produced by the intestinal mucosa in response to the oral intake of nutrients. Insulin secretion from oral glucose intake is greater than insulin secretion from IV glucose, termed the incretin effect [13]. The incretin effect is lower in those with T2DM, largely mediated by the incretin hormone GLP-1. Deterioration of the incretin effect leads to a decreased ability to secrete insulin and hyperglycemia, which may exacerbate insulin resistance [14].
The native GLP-1 peptide is a derivative of the proglucagon gene and is principally synthesized by the L-cells of the ileal mucosa. It is also produced, to a lesser extent, by the preproglucagon (PPG) neurons of the brain [15]. GLP-1 binds to GLP-1 receptor (GLP-1R), a G-protein coupled receptor present in several tissues throughout the body. Native GLP-1 has a short half-life and is degraded in minutes by dipeptidyl-peptidase 4 (DPP-4), mediated by alanine at position 8 [16]. Many GLP-1RAs are modified at this position to provide protection against DPP-4 degradation. GLP-1Rs are distributed widely throughout the body in humans and model organisms, with particular density in the pancreas β-cells, gastrointestinal tract, brain, kidneys, heart, and blood vessels of other organs [17, 18].
Broadly, GLP-1RAs act to increase insulin secretion, decrease glucagon secretion, delay gastric emptying, and induce satiety [14, 19]. Accordingly, GLP-1RAs are prescribed in the treatment of T2DM. GLP-1RAs approved for use in the treatment of T2DM include semaglutide, liraglutide, and dulaglutide. Several randomized controlled trials demonstrated improved cardiovascular outcomes in type 2 diabetics taking GLP-1RAs [20–24]. Accordingly, the American Diabetes Association recommends the use of GLP-1RAs with or without metformin in patients with atherosclerotic cardiovascular disease (ASCVD), chronic kidney disease (CKD), and/or heart failure (HF) [25]. Through enhanced pharmacokinetic profiles, once-weekly GLP-1RA injections, notably semaglutide, are becoming increasingly popular in the treatment of T2DM. Semaglutide acts on the pancreas to reduce both pre- and post-prandial blood glucose concentrations in a glucose-dependent manner primarily by increasing insulin production and inhibiting the secretion of glucagon. Furthermore, it acts on the stomach and the brain to control gastric emptying and feelings of satiety, which aid in the management of metabolic risk factors that contribute to T2DM exacerbation. Compared to the control, T2DM patients treated with semaglutide 1 mg saw a reduction of 29 mg/dL (22%) for pre-prandial glucose, 74 mg/dL (36%) for 2-h postprandial glucose, and 30 mg/dL (22%) for mean 24-h glucose concentration [26]. Several GLP-1RAs have been approved for the treatment of obesity. Recent trials show that overweight or obese patients saw a 14.9% reduction in body weight after taking once-weekly semaglutide 2.4 mg for 68 weeks, compared to a 2.4% reduction in body weight for the control [24]. Similar findings were seen with once-weekly, subcutaneous tirzepatide (5 mg, 10 mg, or 15 mg) [27], demonstrating the utility of GLP-1RAs for obesity management.
Within islet β cells, GLP-1RAs activate adenylate cyclase to increase the production of intracellular cyclic adenosine monophosphate (cAMP) and to start the subsequent signaling cascade (Figure 1) [28]. Enhanced insulin secretion is glucose-dependent via potassium-sensitive adenosine triphosphate (K-ATP) channels mediating K+ efflux, which close with a rise in intracellular ATP concentration from glucose metabolism. GLP-1RAs may also have effects on a transcriptional level via protein kinase A (PKA), phosphorylating cAMP-response element-binding protein (CREB) in the nuclear membrane to enhance preproinsulin transcription [28]. In rodents, GLP-1 has been shown to increase the biosynthesis of glucose transporter 2 (GLUT-2) and glucokinase [29].
Of particular note, GLP-1 interacts with its G-protein coupled receptor, GLP-1R, to exert stimulatory action on adenylyl cyclase. Adenylyl cyclase activation upregulates cAMP production, which exerts its effects on downstream targets via cascade signaling. This can influence several cellular functions, including insulin secretion in pancreatic β islet cells, as depicted in the schematic.
While the mechanisms for GLP-1RAs’ primary insulinotropic effects are clear, such clarity is not apparent for their other effects. Like insulin, glucagon is also inhibited in a glucose-dependent manner. Controversy exists on whether GLP-1Rs exist on pancreatic α cells, with evidence pointing to an indirect effect of GLP-1RAs on glucagon inhibition. GLP-1-induced intra-islet insulin secretion may locally inhibit glucagon secretion. Other mechanisms of glucagon reduction include increased production of somatostatin, a potent glucagon inhibitor, in the delta cells of the pancreas which can exert a paracrine effect on alpha cells. This is supported by studies that show that GLP-1 coadministration with somatostatin receptor antagonists eliminates the glucagon-suppressing effects of GLP-1 [30]. Nevertheless, a 2018 study showed that less than 0.5% of the α cells have GLP‐1R immunoreactivity, supporting a direct α cell effect in a PKA-dependent manner [31]. Electrophysiological measurements showed decreased membrane potential consistent with mathematical models for the inhibition of calcium channels. Inhibition of such calcium channels may, in turn, inhibit the secretion of glucagon-containing vesicles.
Interestingly, higher incidence of diabetic retinopathy (DR) complications was noted in patients taking semaglutide (3.0%) compared to placebo (1.5%) in clinical trials, with greater risk in those with a history of DR [32]. Though the mechanism is not clear, it is suggested that the acute reduction in blood glucose may cause these effects [33]. Glucose, as an osmotically active molecule, may reduce osmotic pressure by bringing water from the interstitium to the vessels, triggering retinal edema. Consequently, DR may be temporarily worsened, and complications may increase from this acute drop in blood glucose, though the long-term effects may prevent further progression of DR. This effect is not observed in other GLP-1RAs and is currently being studied by the drug manufacturers in the FOCUS trial (NCT03811561). As such, the effect of GLP-1RAs on DR should be viewed with caution. A 2023 study combining evidence from a population-based study and Mendelian randomization analyses found a lower incidence of DR in patients treated with GLP-1RAs [34]. Another important association of GLP-1RA use is a higher likelihood of thyroid cancer, with a hazard ratio of 1.58 [35]. A summary of clinically-significant GLP-1RAs described in this text and their clinical uses are listed in Table 1.
Summary of clinically-significant GLP-1RAs
Drug (commercial name) | Administration | Half-life | Hemoglobin A1c effects | Weight-loss effects | Neuroprotective effects (pre-clinical) |
---|---|---|---|---|---|
Semaglutide (Rybelsus) | p.o. | 1 week | High | High | - |
Semaglutide (Ozempic) | s.c. | 1 week | High | High | Yes |
Liraglutide (Victoza) | s.c. | 13 h | High | High | Yes |
Dulaglutide (Trulicity) | s.c. | 5 days | High | Intermediate | Yes |
Terzepetide (Mounjaro) | s.c. | 5 days | High | High | - |
Exenatide (Byetta) | s.c. | 2.4 h | Low | Low | Yes |
Lixisenatide (Adlyxin, Lyxumia) | s.c. | 3 h | Low | Low | Yes |
-: not applicable/no data available
In the gut, GLP-1RAs delay gastric emptying by acting on the enteric nervous system and intestines. This slows the rate of entry of nutrients into the small intestine and their subsequent absorption, thereby affecting post-prandial glucose homeostasis. GLP-1Rs are located throughout the gastrointestinal tract, including parietal cells and on vagal afferents [36]. Mechanistically, delayed gastric emptying is due to cholinergic inhibition of parasympathetic outflow and nitric oxide (NO) release in a cAMP/PKA-dependent manner [37]. Delgado-Aros et al. [38] noted GLP-1 binds to receptors on vagal afferent neurons, which decreased postprandial pancreatic digestive enzyme secretion. This suggests inhibition of vagal cholinergic input into the stomach with a concomitant increase in gastric volume, though the exact mechanism is not clear. Vagal function is critical in delaying gastric emptying, as patients with vagal neuropathy do not observe this [39]. The NO pathway is especially important in endothelial cells, as PKA activates NO synthase (NOS), which can produce more nitric acid and does result in reduced small intestine, large intestine, and colon motility. PKA induction from native GLP-1 phosphorylates a key serine residue in NOS [40]. This promotes reduced contraction and increased relaxation of many parts of the gastric system, including the antrum of the stomach.
In addition to its effects on insulin and glucagon, GLP-1RAs work on the brain for an anorexigenic effect by promoting feelings of satiety and modulating energy homeostasis. In the central nervous system, GLP-1Rs are located in numerous regions and it is suggested that GLP-1RAs act to promote weight loss through distributed effects rather than a specific or localized response [41]. GLP-1R is expressed on cocaine- and amphetamine-regulated transcript (CART)/proopiomelanocortin (POMC) neurons in the hypothalamus, which regulate satiety and termination of meals [40]. Binding of GLP-1 has been reported in the nucleus of the solitary tract (NTS) of the medulla oblongata as well. GLP-1R populations in the nucleus accumbens (ACBs), paraventricular nucleus (PVH), lateral hypothalamus (LHA), and ventral tegmental area (VTA) are involved in food choice, reward, and taste aversion [40]. The local mechanisms of these effects are still under investigation, but GLP-1R co-expresses with proteins known to play an important role in appetite, such as prolactin-releasing peptide and tyrosine hydroxylase [42, 43]. As researchers continue to uncover the many roles of GLP-1, GLP-1R, and synthetic GLP-1RAs in systems beyond the endocrine, potential pharmacologic applications of GLP-1RAs in a variety of pathology are becoming increasingly clear. Specifically, understanding the role of GLP-1 and GLP-1RAs in the neurologic, and by extension, neuro-ophthalmic systems is of particular interest and the focus of this review.
Neurologic role of GLP-1
General anti-inflammatory functions of GLP-1
Beyond its well-established role in glucose control in diabetes mellitus, GLP-1 has increasingly become the target of anti-inflammatory therapies for the management of conditions such as atherosclerosis, nonalcoholic steatohepatitis, nephropathy secondary to diabetes, and neurodegenerative disorders [44]. In general terms, the anti-inflammatory action of GLP-1 contains various mechanisms of action. For example, in the context of vascular anti-inflammatory response pertaining to atherosclerosis, GLP-1RA liraglutide was found to inhibit human plasminogen activator inhibitor type-1 secondary to tumor necrosis factor alpha (TNF-alpha) signaling [45]. Another preclinical study in mice had determined that another GLP-1RA, exendin-4, reduced the development of atherosclerosis and decreased the number of monocytes located on the vascular endothelium, further highlighting the anti-inflammatory role of GLP-1 [46]. This study further demonstrated that exendin-4 treatment additionally reduced the presence of key inflammatory molecules, including TNF-alpha, interleukin 1 beta (IL-1beta), and IL-6, in mice livers [46], providing evidence for potential GLP-1 treatment for inflammation related to steatohepatitis.
Sources and targets of GLP-1 in the brain
GLP-1 and GLP-1R additionally have a substantial presence in the brain. They are endogenously sourced from PPG neurons that are situated caudally in the solitary tract of the brainstem [47, 48]. These neurons produce GLP-1 via posttranslational processing induced by neuroendocrine prohormone convertase [49]. Through studies of mice and rat models, it has been determined that PPG neurons contain projection tracts to various locations on the brain, including primarily the hypothalamus, supraoptic nucleus, and autonomic regions such as the limbic forebrain, portions of the mesolimbic reward system, and other regions involved in learning and memory [47, 50]. These regions contain high densities of GLP-1R, allowing GLP-1 to directly exert its action onto the brain tissue. It should be noted that the cerebral cortex and the cerebellum interestingly lack the expression of the GLP-1R in rat models [51]. Additionally, it is believed that the PPG neurons are not the only source of GLP-1 in the brain, as gut-derived GLP-1 has been implicated in satiety studies to exert its effects on the brain as well [52]. Some studies have demonstrated the ability of gut-derived GLP-1 to cross through the blood-brain barrier and thoroughly perfuse the brain parenchyma, but whether its effects are similar to that of PPG-derived GLP-1 remains to be established [50, 53].
The various locations of GLP-1R in the brain manifest the different neurological and behavioral roles that GLP-1 plays in the central nervous system. Among the most well-understood behavioral mechanisms of GLP-1 in the brain is its ability to restrict feeding behavior through its targeting of the PVH of the hypothalamus and the central amygdala [50, 54]. Functional studies in rat models have demonstrated that injection of GLP-1 has a dose-dependent effect on reducing feeding behaviors [54, 55], providing evidence of its function as a satiety mediator. Additionally, GLP-1R expression in the nucleus ACBs may also contribute to this satiety function, more directly in the context of food-seeking motivated behaviors and reward signaling [56]. Across these studies, the notion of food aversion and nausea through GLP-1 signaling has been implied, but there lacks sufficient evidence to support this claim [56].
Anti-inflammatory and neuroprotective roles of GLP-1
Currently, GLP-1 has been a therapeutic target for its function as an anti-inflammatory mediator as a potential avenue for neuroprotection. Beyond just its function as a satiety mediator, GLP-1 has been demonstrated to exhibit effects on memory and learning, specifically through its action on the hippocampus. In particular, one study demonstrated that hippocampal GLP-1R deficient mice develop deficits in spatial and associative learning [57]. The same study also illustrated that upregulation of GLP-1 via gene transfer was associated with enhanced learning and memory, providing evidence of the role GLP-1 has in hippocampal function [57]. Although the exact mechanism of how this is achieved remains unknown, the authors emphasize the potential ability of GLP-1 to protect the central nervous system [57]. Specifically, it was highlighted that the GLP-1 deficient mice were markedly more prone to developing seizures and hippocampal degradation [57], thus illustrating GLP-1’s importance in preventing these occurrences.
Furthermore, it is well-established that glia have a substantial function in the inflammatory response of the central nervous system and thus are highly implicated in neurodegenerative diseases. In fact, it has been experimentally determined that amoeboid microglia express GLP-1 immunoreactivity, thus providing evidence of their interactions with GLP-1 [58]. In addition to this, both astrocytes and microglia together can bind GLP-1 [58]. A study demonstrated that GLP-1, via cAMP-systems mediated by astrocytes, inhibits the expression of IL-1beta, an inflammatory molecule that is highly implicated in cell death, brain injury, and neurodegeneration [58]. Additionally, IL-1beta is thought to be involved in amyloid precursor synthesis and the production of other cytokines involved with neurodegenerative disorders [58].
As such, GLP-1 has been studied as a potential therapeutic for the treatment and management of high-impact neurodegenerative disorders, namely Alzheimer’s disease (AD) and Parkinson’s disease (PD). The link to AD was previously implicated through its association with T2DM, where insulin receptors and signaling were found to be similarly desensitized between both conditions [59]. There are many potential modalities in which GLP-1 can serve a role in protecting against the progression of AD. One study has shown that induced neuropathy can be reversed via the administration of GLP-1 and its agonist, exendin-4 [60]. Their results demonstrate that GLP-1, through an unknown mechanism, can preserve axonal and neurofilament structure despite the induction of progressive neurotoxicity, thus illustrating neuroprotective properties that can be leveraged against disorders in the central nervous system [60].
With this knowledge, studies are being conducted to determine the effects of existing GLP-1 drugs for diabetes on the central nervous system. One such study by McClean and colleagues [61] observed that liraglutide can cross the blood-brain barrier in substantial amounts and can prevent deficits in the water maze task performance and object recognition in AD model mice. In addition to behavioral preservation, the study also determined that the number of synapses remained unchanged and the number of plaques and amyloid oligomers decreased, all physical indications against neurodegeneration [61]. Lastly, this study highlighted the decrease in brain inflammation and marked increase in glial cell activation [61], again illustrating the anti-inflammatory and neuroprotective properties of GLP-1. Additionally, as part of the EVOKE trials, there are currently phase III trials conducted by Nordisk [62] investigating the use of semaglutide in the treatment of patients with early-stage AD [63]. Use of GLP-1RAs in an AD mouse model has also shown a neuroprotective role in promoting a reduction in glutamate-induced currents [64]. It is well known that increased glutamate levels are associated with increased neuronal activity, leading to glutamate-induced excitotoxicity via reactive oxygen species (ROS) production [65, 66]. By mitigating the impact of glutamate on neuronal excitation, excitotoxicity-induced neuronal cell death can be reduced. In animal models, pre-treatment of neurons has shown a decrease in neuronal cell death caused by glutamate excitotoxicity as well [65]. Studies have also shown the potential of the DPP-4 inhibitor alogliptin in increasing production of brain-derived neurotrophic factor (BDNF), leading to decrease toxic release of glutamate [67].
These properties do not only extend to the protection against AD. Previous studies have shown that gliosis near substantia nigra neurons may contribute to the progression of PD via microglial-mediated injury [68]. This injury is potentially mediated via pro-inflammatory cytokines, particularly TNF-alpha and IL-1beta [69]. As mentioned previously, GLP-1 exerts effects on glial cells to inhibit these cytokines [58]. A study conducted on PD model mice shows that the administration of exedin-4 both prevented microglial activation in the substantia nigra pars compacta and significantly reduced the neuronal loss in that region, as well as with striatal dopaminergic nerve fibers [70]. The study also shows that markers of neuronal cell stress and apoptosis, namely metalloproteinase-3, were significantly reduced upon the administration of GLP-1 [70]. Again, this study highlights the potential therapeutic capability of GLP-1 as a neuroprotective agent, particularly through its anti-inflammatory mediatory ability.
Neuro-ophthalmo-protective effects of GLP-1
The ophthalmic system, in many ways, serves as an extension of the brain. This is particularly true for the retina, as it originates from neural ectoderm and connects to the brain by the optic nerve, which shares the meningeal layers of the brain. Much like in the brain, inflammation is a common feature in several ocular pathologies, particularly those relating to the retina. It is well known that inflammation is linked with breakdown of the blood-retinal barrier, which results in vascular dysfunction and edema. Given the principal anti-inflammatory effect of GLP-1 in the central nervous system elucidated in previous sections, research on the role of GLP-1 in the neuro-ophthalmic circuit shows a similar beneficial promise. Broadly, studies suggest GLP-1RAs exert their neuroprotective effects in the retina by mediating reduced glutamate excitotoxicity, decreased neuroinflammation, prevention of retinal ganglion cell (RGC) loss, promotion of vascular integrity, promotion of an anti-oxidative environment, and maintenance of glial cells (i.e. Müller glia, Figure 2) [71–77].
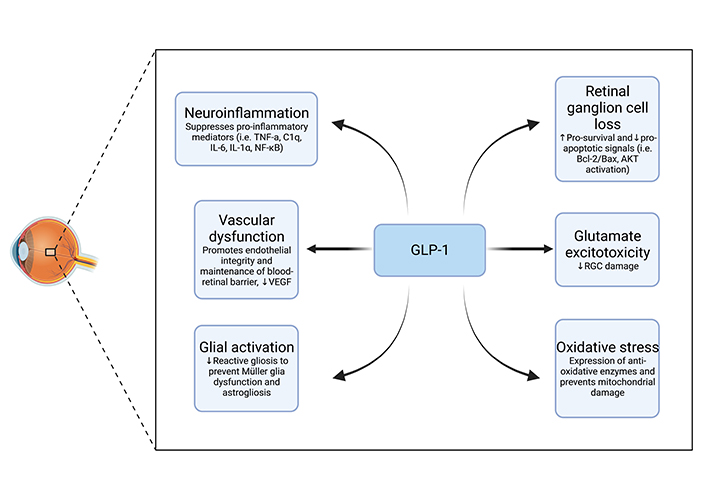
Schematic of GLP-1 neuroprotective mechanisms of action in the retina. VEGF: vascular endothelial growth factor; NF-κB: nuclear factor kappa B; Bcl-2: B-cell leukemia/lymphoma 2 protein; Bax: BCL-2-associated protein x; AKT: protein kinase B
GLP-1 exerts neuroprotective effects via several mechanisms of action. GLP-1 prevents neurodegeneration via upregulation of GLUT, thus reducing the potential for glutamate excitotoxicity. GLP-1 downregulates the production of pro-inflammatory cytokines such as TNF-alpha, IL-1, and IL-6, which results in a decrease in neuroinflammation. Additionally, GLP-1 prevents RGC loss via the promotion of neurogenesis. GLP-1 exerts regulatory functions on retinal capillaries and maintains the stability of the blood-retinal barrier, preventing foreign substances from negatively affecting vital retinal neurons. Oxidative stress is also reduced by the structural maintenance of mitochondria exerted by GLP-1 and by its upregulation of anti-oxidative molecules. Lastly, GLP-1 induces the maintenance of glial cells, such as Müller glia, by downregulating the reactivity of astrocytes.
GLP-1 and DR
Given GLP-1’s role in diabetes management, pre-clinical and clinical research regarding the neuro-ophthalmologic effects of GLP-1 is mainly centered around DR. The retina is particularly susceptible to inflammation due to the high bioavailability of glucose and its subsequent oxidation. In diabetic patients, higher glucose levels and greater glycemic variability promote a pro-inflammatory state, which leads to defects in microvasculature characteristic of DR [78]. As the disease progresses, hypoxia may induce the release of angiogenic inflammatory cytokines (i.e. VEGF), leading to the aberrant angiogenesis seen proliferative DR. Emerging evidence indicates that lipid traits may have a role in DR as well, though work to understand this mechanism is still being conducted [79].
Several studies posit an anti-inflammatory and anti-apoptotic role of GLP-1 in both preventing and reducing the progression of DR; in diabetic rodents, Fan and colleagues [77] demonstrated that intravitreal exedin-4 injection reduced retinal cell loss, maintained Bcl-2/Bax ratios, whose imbalance is associated with retinal cell apoptosis, prevented activation of pro-apoptotic AKT, and prevented excess reactive gliosis. Through a reduction in extracellular glutamate and an increase in pro-survival signaling, Hernández et al. [80] were able to show how both topical and systemic liraglutide administration prevented retinal degeneration in db/db mice. Moreover, retinal protection was achieved without affecting systemic blood glucose levels in this study, suggesting the protective effects of GLP-1 are independent of blood glucose or insulin [80]. NF-κB, pro-inflammatory cytokines, and VEGF were also shown to be blunted with topical GLP-1 administration in diabetic mice [81].
Most recently in November 2022, Wei and colleagues [82] evaluated mice with T2DM on a high-sugar and high-fat diet for changes in retinal structure following GLP-1RA treatment. While this study did show lowered blood glucose levels, GLP-1RA treatment improved retinal thickness, morphology, and vasculature [82]. Importantly, they demonstrated that GLP-1 protected the blood-retinal barrier. While the mechanism is not yet proven, there is support for the idea that GLP-1 attenuates the ras homolog gene family, member A (RhoA)/Rho-associated protein kinase (ROCK) signaling pathway, whose activation is known to reduce levels of occludin, claudin-5, and zonula occludens-1 (ZO-1), as well as promote disruption of endothelial tight junctions. Mice with DR exhibited elevated levels of ROCK and phosphorylated myosin light chain (p-MLC) originally, but decreased levels of both proteins were seen with GLP-1 involvement [82]. Several studies point to a protective role of GLP-1RAs on retinal pigment epithelium (RPE) cells, which form the blood-retinal barrier and are vulnerable to inflammation [83, 84]. GLP-1R activation has been suggested to enhance the ability of RPE cells to counteract inflammation and oxidative stress, as RPE cells exposed to TNF-alpha and high glucose show enhanced survival characteristics following treatment with exenatide [83, 84].
In RGCs, a diverse group of neurons found in the inner retinal surface with long axons that connect to the brain to relay visual information, neurodegeneration is also seen and, in fact, may precede changes in microvasculature in DR [85]. RGCs are vulnerable to oxidative and inflammatory stressors and have been shown to be thinned in hyperglycemic conditions [86–88]. With high levels of mitochondria, mitochondrial integrity is a critical feature of RGCs, accordingly making mitophagy a critical physiologic pathway. In RGC cell lines and in vivo, Zhou and colleagues [89] showed hyperglycemia decreased the viability of RGCs and increased mitophagy beyond the basal levels required for homeostasis. Application of liraglutide reduced mitochondrial damage by attenuating mitophagy via the protein phosphatase and tensin homolog-induced kinase 1 (PINK1)/Parkin pathway, the most well-known pathway for mitophagy (Figure 3). In vitro, Ma and colleagues [90] also showed liraglutide to reduce H2O2-related damage in RGCs through inhibition of mitophagy induction, primarily by reducing ROS and maintaining mitochondrial membrane potential. Liraglutide has also been seen to inhibit tau-hyperphosphorylation in DR, thus protecting RGCs from degeneration [91].
As cells composed of high levels of mitochondria, RGCs maintain a balanced microenvironment via the tight regulation of mitochondria integrity through the process of mitophagy—a PINK/Parkin-dependent pathway. However, when exposed to external stressors such as hyperglycemia, complex dysfunction can lead to increased levels of this physiological process. Employment of the GLP-1RA liraglutide has been shown to diminish this damaging effect through upstream modulation of the PINK1/Parkin pathway to effectively counteract high glucose-induced cell dysfunction.
Another mechanism is that GLP-1 may reduce RGC loss indirectly by preventing Müller cell gliosis. Müller cell gliosis, marked by an increase in glial fibrillary acidic protein (GFAP), can cause excitotoxicity-induced cell death of RGCs [92]. Fan et al. [77] showed exedin-4 reduced GFAP in mice, suggestive of reduced gliosis. Geniposide similarly inhibited Müller cell activation in vitro and in vivo [93]. Taken together, various mechanisms may mediate a neuroprotective role of GLP-1 in the context of DR. Interestingly, no preclinical studies evaluate the mechanism between DR and semaglutide, which is the only GLP-1RA to show high levels of DR in trials. These mechanisms necessitate further investigation into the pathways of GLP-1RA involvement in the clinical course of DR to learn if they hold substantial value beyond a pre-clinical setting [91].
GLP-1 and glaucoma
Glaucoma is a neurodegenerative disease characterized by a progressive decline in RGCs which reside in the optic nerves [71, 94, 95]. It is the leading cause of blindness, with key risk factors of age, genetics, and high intraocular pressure. A recent case-control study of 1,961 new GLP-1RA patients showed that use of GLP-1RAs significantly reduced the risk of glaucoma, with a hazard ratio of 0.56 [94]. Since, studies have attempted to elucidate the mechanisms governing this reduction and further identify the clinical utility of GLP-1RAs, but much must be inferred from preclinical studies in diabetic models.
Like in DR, inflammation is critical in the pathophysiology of glaucoma. Accordingly, many of the neuroprotective mechanisms outlined in the discussion of DR have implications for glaucoma as well. In CD11b+ CD11c+ cell lines, Sterling and colleagues [73] showed conditions of glaucoma increased retinal inflammation and astrogliosis. Subsequently, reduced inflammation was achieved via administration of NLY01, a GLP-1RA, resulting in lower levels of RGC death and astrogliosis. Pro-inflammatory cytokines from activation of microglia can lead to the formation of A1 astrocytes, which are implicated in the pathogenesis of glaucoma and have diminished ability to phagocytose and poorer synapse support [96, 97]. Lower levels of the A1 proinflammatory form of astrocytes were seen following administration of NLY01 [73]. Thus, GLP-1RA mediates inflammation to prevent formation of neurotoxic astrocytes which cause RGC death.
The importance of glial cells largely in the maintenance of RGCs and overall optic nerve integrity cannot be understated, as glial cells collectively play several important roles in regulating the retinal microenvironment [98]. In addition to supporting the delivery of growth factors and nutrients to RGCs, glial cells also play a primary role in removing excess glutamate from the extracellular space to prevent RGC damage associated with glutamate excitotoxicity [91, 98, 99]. As in the pathophysiology of DR, RGCs are particularly vulnerable to oxidative stressors in glaucoma. GLP-1RAs thus prevent the occurrence of retinal damage associated with oxidative-stress-induced activation of glial cells and collectively foster a healthy retinal microenvironment by virtue of preventing cellular overexertion [94, 100]. Specifically, Müller cells are important in the pathogenesis of glaucoma by providing support across the retinal span. High levels of GFAP are also noted in glaucoma; given the known effects of GLP-1RAs on GFAP in the models of DR, it is plausible for a direct effect of GLP-1RAs on Müller cells activation in the context of glaucoma. As future studies unravel the link between GLP-1 and glaucoma, the full breadth of direct and indirect effects leading to RGC neuroprotection must be considered given the versatility of the peptide.
GLP-1 and IIH
The versatility of GLP-1 applications is also showcased in the context of IIH. IIH is driven by an increase in intracranial pressure that can present as severe visual loss to debilitating headaches due to compression of the optic nerve and papilloedema [101]. GLP-1R has been densely found in the choroid plexus, suggesting the possibility it may play a role in sodium transport and cerebrospinal fluid (CSF) production [102]. In rodents, Botfield and colleagues [103] demonstrated that exendin-4 administration reduced intracranial pressure in hydrocephalic female rats. Furthermore, sodium and potassium-dependent ATP activity, a key marker of CSF secretion, was reduced [103]. These positive results prompted the first clinical trial of exenatide in treating patients with IIH. Compared to control, significant reductions in intracranial pressure were seen acutely at 2.5 h and 24 h, but also at 12 weeks [101]. Furthermore, the results illustrated a significantly reduced incidence of headaches and visual deficits [101]. Current literature suggests that this potential in lowering intracranial pressure is associated with its correlation in reducing CSF secretion through a protein kinase signaling pathway [104]. These results show much clinical promise, as the magnitude of improvement in patients is comparable to the standard of care, acetazolamide. However, acetazolamide is poorly tolerated in patients while GLP-1RAs are typically better tolerated, with only transient nausea as the predominant adverse effect [105].
Conclusions
In this review, multiple considerations that have recently contributed to the demand for advancements in the field of neuro-ophthalmology were identified—namely, the increasing morbidity of neuroophthalmological disease processes, physician workforce shortages, and an increasing geriatric population. Together, this multifactorial dilemma stipulates the necessity for the field to investigate novel pharmaceuticals and to extend the therapeutic utility of well-researched drugs already used in clinical practice. Regarding the latter resolution, this paper introduced the use of GLP-1RAs—a drug class traditionally used in the treatment and management of T2DM and obesity—as a potential avenue for the treatment of neurodegenerative diseases and neuropathologic conditions alike due to the drug’s extensively cited neuroprotective effects.
Specifically, this paper delved into an overview of GLP-1’s role at the physiological level as a modulator of the “incretin effect”, which is an important hormonal pathway whose dysregulation was explained to be implicated in the pathogenesis of T2DM. Particularly, the reduction of GLP-1 release over time has been an observed feature of T2DM development, as GLP-1 reduces glucagon secretion and hepatic gluconeogenesis while promoting insulin secretion, thereby effectively minimizing hyperglycemia [106]. Further, it was explained that the appetite-reducing effects promoted by GLP-1R stimulation effectively reduce caloric intake by inducing satiety and delaying gastric emptying, making it a prime target to counter hyperglycemia and overeating [107]. Taken altogether, these mechanisms explain the clinical use of GLP-1RAs as beneficial treatment options for T2DM and obesity.
However, as explained above, GLP-1Rs are not only localized to organs involved in the glycemic response, such as the pancreas and the liver, but are also expressed in various regions of the brain through neurons and glial cells [108]. Consequently, this vast expression of GLP-1R has enabled researchers and clinicians to explore potential therapeutic uses of GLP-1RAs in neurologic systems and their implications in sub-neurologic systems, principally the neuro-ophthalmic system. Among the notable mechanisms of action common to why this peptide has such powerful neuroprotective effects is chiefly the anti-inflammatory functions of GLP-1. With regards to neurodegenerative diseases such as AD and PD, the literature has demonstrated the inhibitory effects of GLP-1 on pro-inflammatory cytokines such as TNF-alpha and IL-1beta—two notorious small molecules identified in the microglial-mediated injury observed in said diseases processes. These effects are currently being evaluated in clinical trials.
Moreover, these anti-inflammatory properties also extend to the neuro-ophthalmic circuit, which is embryonically related to the central nervous system and is thus similarly afflicted by pathologies driven by inflammation. Given the established clinical use of GLP-1RAs for the treatment of T2DM, preclinical and clinical research was centered largely around DR, which suggested the neuropathologic protective effects of GLP-1 are due to downregulation of NF-κB, proinflammatory cytokines such as TNF-alpha and IL-1beta, and VEGF. Furthermore, this review highlighted the importance of maintaining the integrity of RGCs, a critical group of neurons that integrate the central nervous system with the visual apparatus which are an area of dysfunction in both DR and glaucoma. Thus, as neurons are particularly prone to inflammatory and oxidative stress secondary to hyperglycemic conditions, the role of GLP-1 in regulating the retinal microenvironment is crucial for the protection and homeostatic regulation of RGCs. Neuroprotective effects of GLP-1 were also seen in other cells in the retinal microenvironment, including RPE and glial, including astrocytes and Müller cells.
Finally, the role of GLP-1 in CSF secretion was overviewed because recent literature points to its clinical employment in treating IIH. Although not directly related to inflammation due to a physiologic change in the microenvironment, GLP-1RAs have the potential to replace current standard of care treatment for IIH and can ultimately contribute to improved patient outcomes in the field of neuro-ophthalmology. Therefore, the aforementioned novel applications of GLP-1RAs all have the potential to bolster treatment and management options for the field as a whole, and new research continues to illuminate the physiological mechanisms behind this observed neuroprotective effect.
Abbreviations
AD: |
Alzheimer’s disease |
cAMP: |
cyclic adenosine monophosphate |
CSF: |
cerebrospinal fluid |
DPP-4: |
dipeptidyl-peptidase 4 |
DR: |
diabetic retinopathy |
GFAP: |
glial fibrillary acidic protein |
GLP-1: |
glucagon-like peptide 1 |
GLP-1R: |
glucagon-like peptide 1 receptor |
GLP-1RA: |
glucagon-like peptide 1 receptor agonist |
IIH: |
idiopathic intracranial hypertension |
IL-1beta: |
interleukin 1 beta |
NF-κB: |
nuclear factor kappa B |
NO: |
nitric oxide |
PD: |
Parkinson’s disease |
PINK1: |
protein phosphatase and tensin homolog-induced kinase 1 |
PKA: |
protein kinase A |
PPG: |
preproglucagon |
RGCs: |
retinal ganglion cells |
RPE: |
retinal pigment epithelium |
T2DM: |
type 2 diabetes mellitus |
TNF-alpha: |
tumor necrosis factor alpha |
VEGF: |
vascular endothelial growth factor |
Declarations
Author contributions
Sohum S, AP, MF, MM, AR, RS, and Siddharth S: Conceptualization, Data curation, Formal analysis, Investigation, Methodology, Project administration, Resources, Software, Supervision, Validation, Visualization, Writing—original draft. Sohum S, AP, MF, MM, AR, RS, Siddharth S, and BLW: Writing—review & editing.
Conflicts of interest
The authors declare that they have no conflicts of interest.
Ethical approval
Not applicable.
Consent to participate
Not applicable.
Consent to publication
Not applicable.
Availability of data and materials
Not applicable.
Funding
Not applicable.
Copyright
© The Author(s) 2023.