Abstract
The glucocorticoid receptor alpha (GRα), a vital component of the ancient glucocorticoid (GC) signaling system, is essential for vertebrate survival. It regulates fertility, fetal development, organ function, vascular and neural integrity, metabolism, immune responses, and stress adaptation. While GRα’s anti-inflammatory properties have been acknowledged since the mid-20th century, its crucial role as the master regulator of homeostatic corrections in both health and critical illness has only recently come to light. In critical illness, GRα facilitates a seamless transition through three essential phases of homeostatic correction. Initially, in the Priming Phase, it activates immune responses and mobilizes energy reserves to defend against stressors like infection and injury, enhancing glucose metabolism, supporting mitochondrial function, and strategically deploying immune cells to areas of damage. Next, during the Modulatory Phase, GRα fine-tunes inflammatory responses, manages oxidative stress, regulates vascular tone, and maintains cellular integrity. Finally, in the Restorative Phase, GRα plays a crucial role in resolving inflammation, initiating tissue repair, supporting cellular regeneration, facilitating debris clearance, and reestablishing anatomical and physiological balance for long-term recovery. GRα coordinates complex molecular interactions, including co-regulation with pro-inflammatory transcription factors, ensures mitochondrial stability, and metabolic balance under stress. However, depleted bioenergetic and micronutrient reserves in critically ill patients can impair GRα’s capacity, increasing morbidity and mortality risks. This review highlights the need to reassess current GC treatment strategies and integrate micronutrient support to optimize GRα function. Such an approach could strengthen immediate immune defenses, enhance long-term recovery, reduce GC dose and duration, and minimize adverse effects.
Keywords
Glucocorticoid, glucocorticoid receptor, homeostatic corrections, critical illness, evolution, general adaptation syndromeIntroduction
This review presents an updated and expanded synthesis of selected content from three previously published textbook chapters [1–3]. It integrates new insights into the evolutionary origins of the glucocorticoid (GC) receptor (GR) and its central role in the pathobiology of critical illness. By exploring GR alpha’s (GRα’s) multifaceted functions, the review emphasizes its profound influence throughout each disease progression and resolution phase. Key sections have been meticulously revised to provide a more comprehensive and detailed summary of our current understanding, reflecting recent advances in the field. This updated perspective aims to deepen the appreciation of GRα’s regulatory impact, from its foundational evolutionary role to its critical importance in managing homeostasis during illness. The GC signaling system, mediated by the GR, has played an essential role in vertebrate evolution and survival, facilitating precise adaptations to environmental stressors, regulating metabolism, and maintaining immune homeostasis. Despite its ancient origins, the full significance of this system began to emerge only in the early 20th century, following the discovery of cortisol’s therapeutic efficacy in treating inflammatory diseases [4]. This breakthrough represented a pivotal advancement in medicine. However, the prevailing focus on GCs as anti-inflammatory agents has often overshadowed their broader and more complex biological roles, which extend well beyond inflammation control and are fundamental to systemic adaptation.
Before exploring the complex role of the GC-GR system in orchestrating homeostatic corrections during critical illness, this review will first consider its evolutionary history and foundational significance in the development of vertebrates, including humans. This historical perspective provides essential context for understanding how the GC-GR system has evolved to meet the challenges of critical illness and restore balance during severe physiological stress.
The evolutionary history of the GC-GR system
The development of homeostatic correction mechanisms, particularly those involving the GC and GR network and their interactions with molecular pathways, has been driven by organisms’ need to survive and adapt to environmental challenges [3, 5]. The GR is essential for life, as multiple GR knockout mouse models show [6]. Steroid signaling pathways, including those mediated by GCs, arose in early jawed vertebrates during the Paleozoic era (specifically around the late Ordovician—Silurian transition) around 450 million years ago as part of the gradual emergence of the hypothalamic-pituitary-adrenal (HPA) axis in vertebrates [7]. The modern GR is the product of hundreds of millions of years of evolutionary history involving ancestral nuclear receptors, gene duplications, natural selection acting on subtle changes in receptor-ligand interactions, and the integration of dietary vitamins (vitamins C, D, and thiamine) [2] into more specialized endocrine pathways.
Interestingly, the GR, nuclear factor-kappa B (NF-κB), and the mechanisms of hemostasis and inflammation also have deep evolutionary roots, tracing their origins to lineages predating the Cambrian explosion. These early signaling systems allowed vertebrates to regulate metabolism and respond to environmental stressors, both essential for survival (Table 1) [5, 8–11]. The co-evolution of the GR with the immune system has improved its capacity to control inflammation and maintain hemostasis, ensuring a vital balance between immune defense and tissue protection. This regulatory equilibrium is illustrated by the interaction between GR and pro-inflammatory transcription factors (TFs) such as NF-κB and activator protein 1 (AP-1) [11]. Over time, the GC-GR system has evolved to respond to a wide range of stressors, including infections, injuries, psychological stress, and metabolic demands.
The evolutionary development of homeostatic corrections
Number | Stage | Description | Key points | Approximate timeline | Reference |
---|---|---|---|---|---|
1 | Ancient origins of steroid signaling | Evolution of steroid signaling pathways to regulate metabolism and stress responses in early vertebrates. These pathways allow organisms to manage energy resources and respond to environmental changes. |
| Approximately 450–500 million years ago | [8] |
2 | Co-evolution with immune, inflammatory, and hemostatic responses | GR co-evolved with the immune system to regulate inflammation and prevent tissue damage. Hemostasis and inflammatory mechanisms evolved alongside, underscoring their interconnected roles. |
| Approximately 400–450 million years ago | [11] |
3 | Adaptation to diverse stressors | GR system evolved to manage a wide range of stressors, including infections, injuries, psychological, and metabolic stress. |
| Approximately 300–350 million years ago | [5] |
4 | Integration with mitochondrial function | GR co-evolved with mitochondrial function, reflecting the role of energy production in stress response. Mitochondria contain GREs in mtDNA. |
| Approximately 1.5–2 billion years ago (mitochondria origin), integration with GR: approximately 400 million years ago | [9] |
5 | Essential micronutrients and antioxidant systems | GR-mediated corrections rely on micronutrients and antioxidants incorporated into stress responses as organisms evolved more complex diets and metabolic systems. |
| Approximately 400 million years ago | [10] |
This table was generated with the assistance of AI using GPT-4. AP-1: activator protein 1; GR: glucocorticoid receptor; GREs: glucocorticoid response elements; mtDNA: mitochondrial DNA; mtGREs: mitochondrial GREs; NF-κB: nuclear factor-kappa B
This evolutionary narrative is further enriched by the integral role of mitochondria, which originated from ancestral bacteria through symbiotic relationships during the same evolutionary period and became essential to the cellular energy strategies underpinning GR-mediated stress responses. The co-evolution of GR and mitochondria highlights their critical collaborative roles in enhancing cellular bioenergetics and systemic homeostasis under stress conditions, a testament to the intricacies of physiological adaptations across millions of years [3, 9]. GRα regulates mitochondria, as GRs are present within them, and glucocorticoid response elements (GREs) have been identified in the mitochondrial genome [2, 12]. During evolution, organisms began tapping into environmental sources of vitamins and minerals for essential biochemical reactions, such as energy production and enzyme function. As certain proteins acquired the ability to bind and utilize these cofactors, they became more efficient and adaptable, providing a selective advantage. Over countless generations, these cofactors became vital components of hormone synthesis and signaling pathways, ultimately shaping modern endocrine systems.
Despite this extensive evolutionary history, the body’s stress response was not systematically studied until the 20th century. This led to groundbreaking insights into systemic inflammation and its progression to organ failure [13].
Brief history of the discovery of GCs and advancements in knowledge
The discovery of cortisone in the late 1940s, particularly for its potent anti-inflammatory effects in treating rheumatoid arthritis, marked a revolution in medicine [14]. Notably, while the risks of administering GC treatment without proper tapering were recognized early on, this crucial consideration remains overlooked in many randomized trials [2]. Since then, GC treatment has been essential in managing multifactorial inflammation; however, its biological significance extends well beyond anti-inflammatory effects. Identifying the GRα in the late 1960s and early 1970s significantly advanced our understanding of GC mechanisms. By the 1980s and 1990s, it became evident that the GC-GRα complex is crucial for transcriptional regulation, underscoring the broader influence of GCs on cellular functions [15]. For further historical context, readers are encouraged to review a comprehensive history of corticosteroid treatment in critically ill patients, which details how scientific advances have shaped treatment protocols [16]. A recent review focuses on the 75-year journey with GCs and the growing understanding of GRs [17].
GC-GR system: master regulator of homeostatic corrections
Over the past two decades, the GR, particularly the GC-GRα system, has emerged as a key regulator of homeostasis by integrating and coordinating signals across multiple pathways. These pathways include stress response, inflammation, hemostasis, immune regulation, cellular bioenergetics, metabolic adaptation, circadian rhythm regulation, neural signaling, mitochondrial function, and tissue repair and regeneration. Through this intricate integration, the GR fine-tunes physiological adjustments during both health and disease progression and resolution, aiding the body in maintaining stability under physiological and pathological conditions [18, 19]. This evolving perspective challenges the traditional view of the GC-GRα system as merely anti-inflammatory, emphasizing its essential role in broader homeostatic corrections—especially in critical illness, where its diverse and extensive actions are frequently underappreciated.
The regulatory functions of GRα have evolved to optimize survival and reproduction, making the GRα system indispensable for vertebrate evolution and essential in both male and female physiology. GRα plays a crucial role in fertility [20], establishes the cellular environment necessary for normal uterine biology, and regulates placental development through uterine-specific actions. It is also vital for all fetal organs’ structural and functional maturation [21–23], influencing the expression of nearly 4,000 genes [24]. The profound importance of the GRα system in reproductive health and developmental processes ensures survival and proper function throughout life. Beyond its foundational role in initiating life, GRα maintains physiological homeostasis, supports organ function, and enables the body to respond to stress and sustain health. The GC-GRα signaling pathway influences nearly every physiological system and upholds vascular and neural integrity. It regulates the function of all organs (Table 2) and circulating cells (Table 3), covering both immune and non-immune cell types. By orchestrating coordinated and precise responses to stress, GRα is crucial for preventing or resolving multi-organ failure in critical illness [1, 19, 25].
Roles of GRα in the regulation of organ and system functions
Organ/System | GRα regulation |
---|---|
Immune system | Modulates innate and adaptive immunity, ensuring a balanced immune response. Regulates macrophages, neutrophils, and dendritic cells, guiding pathogen and injury responses. Controls T and B cell activation, proliferation, and cytokine production. Upregulates GILZ and annexinA1 to mitigate inflammation, facilitate macrophage clearance of apoptotic cells, and restore immune homeostasis. |
Lymphatic system | Regulates lymphatic endothelial cell function, maintaining vessel integrity, permeability, and contractility. Enhances lymph flow and immune surveillance by modulating transporter and receptor expression, supporting effective immune response and antigen transport. |
Central nervous system | Regulates the HPA axis, managing stress responses and restoring homeostasis. Modulates serotonergic and dopaminergic systems, affecting mood, cognition, and behavior. Influences synaptic transmission and neuronal plasticity, which are critical for memory and executive function. Supports brain metabolism by ensuring glucose availability during stress and recovery. |
Peripheral nervous system | Modulates stress responses, influencing pain perception and inflammation. Supports nerve regeneration and repair, promoting recovery post-injury. Regulates the autonomic nervous system, maintaining heart rate, blood pressure, and gastrointestinal motility under stress. |
Endocrine system | Influences cortisol production via the HPA axis, coordinating metabolic and immune responses. Regulates glucose homeostasis, thyroid, and reproductive hormones, ensuring hormonal balance during stress. Supports growth hormone balance and RAAS to maintain systemic equilibrium. |
Cardiovascular system | Maintains vascular tone, blood pressure, and heart function. Promotes vasodilation through eNOS expression and prevents excessive vasoconstriction. Regulates cardiac glucose metabolism and ion channel function, preserving energy and rhythm. Mitigates oxidative stress and inflammation, preventing cardiovascular complications. |
Endothelium | Preserves endothelial barrier integrity and glycocalyx, reducing inflammation and vascular permeability. Modulates nitric oxide production for vascular tone and supports angiogenesis and the repair of damaged vessels. Protects against oxidative stress, preventing atherosclerosis, hypertension, and dysfunction. |
Lungs | Regulates immune responses, mitigating inflammation and preventing tissue damage. Supports tissue repair, bronchodilation, and surfactant production, ensuring airway integrity and efficient gas exchange. Preserves vascular integrity and facilitates recovery during conditions like asthma, COPD, and ARDS. |
Kidneys | Regulates electrolyte balance and fluid homeostasis by modulating sodium channel expression in renal tubules. Maintains blood pressure and overall fluid balance through precise control of sodium and water reabsorption. |
Liver | Enhances glucose metabolism through gluconeogenesis and glycogen storage. Regulates lipid metabolism, detoxification, and acute phase protein synthesis, boosting immune defense and managing inflammation. Works with IL-6 to coactivate the acute phase response during stress. |
Gastrointestinal tract | Maintains gut integrity by controlling inflammation and promoting tissue repair. Modulates the gut microbiome, immune responses, motility, and nutrient absorption. |
Pancreas | Regulates insulin production and glucose homeostasis by modulating pancreatic beta, alpha, and delta cells. Ensures proper hormone secretion during stress and fasting to maintain glucose balance. |
Adipose tissue | Promotes lipolysis and regulates lipid storage and mobilization, ensuring energy availability during stress or fasting. |
Muscle | Balances protein breakdown and synthesis, supporting gluconeogenesis and muscle repair. Modulates inflammation to preserve muscle function and resilience during stress and recovery. |
Bone | Regulates bone remodeling by balancing osteoblast and osteoclast activity, maintaining bone density and structural integrity. |
Skin | Modulates inflammatory responses, preventing excessive cytokine production. Protects the skin from infections and environmental stressors, ensuring immune defense and tissue integrity. |
This table outlines some of the essential functions of GRα in various organs and systems under normal conditions. GRα plays a critical role in maintaining homeostasis, modulating stress responses, and ensuring the proper functioning of vital physiological processes. This table was generated with the assistance of AI using GPT-4. ARDS: acute respiratory distress syndrome; COPD: chronic obstructive pulmonary disease; eNOS: endothelial nitric oxide synthase; GILZ: glucocorticoid-induced leucine zipper; GRα: glucocorticoid receptor alpha; HPA: hypothalamic-pituitary-adrenal; RAAS: renin-angiotensin-aldosterone system
Roles of GRα in the regulation of circulating cells
Cell type | Brief description of GRα’s role in the regulation of circulating cells |
---|---|
T cells | Modulates T cell function by inhibiting pro-inflammatory cytokines (e.g., IL-2, IFN-γ), promoting Treg differentiation, and reducing effector T cell proliferation. Ensures suppression of excessive immune responses and maintenance of immune tolerance. |
B cells | Suppresses B cell activation and differentiation into plasma cells, reducing antibody production. Influences B cell survival and regulates cytokines like IL-10, contributing to balanced immune responses. Like IL-10, which further influences immune responses. |
Monocytes/Macrophages | Regulates monocyte-to-macrophage transition and polarization into pro-inflammatory (M1) or anti-inflammatory (M2) phenotypes. Suppresses inflammatory mediators (e.g., TNF-α, IL-6) while enhancing phagocytosis and tissue repair. |
Dendritic cells | Modulates DC maturation and antigen-presenting capacity, reducing T cell activation. Promotes immune homeostasis, particularly during chronic inflammation. |
NK cells | Influences NK cell cytotoxic activity, reducing their ability to target virus-infected or tumor cells. Modulates cytokine production (e.g., IFN-γ), shaping overall immune responses. |
Eosinophils | Suppresses eosinophil activation and reduces pro-inflammatory cytokine and chemokine production, mitigating allergic inflammation. Decreases eosinophil survival and migration, controlling inflammation in asthma and allergic conditions. |
Erythrocytes | Regulates erythropoiesis by influencing erythropoietin production and red blood cell maturation. Impacts hemoglobin synthesis and oxygen transport capacity, particularly during stress. |
Platelets | Modulates platelet activation and aggregation by regulating surface receptor expression. Maintains hemostatic balance, preventing excessive clot formation during inflammation. |
Endothelial progenitor cells | Influences mobilization and differentiation of EPCs, which are vital for vascular repair and regeneration. Regulates factors involved in endothelial function and vascular integrity during injury or stress. |
This table summarizes the roles of GRα in regulating immune and non-immune circulating cells, highlighting its pivotal role in maintaining immune homeostasis, supporting vascular repair, and ensuring balanced responses during stress and inflammation. Each cell type is accompanied by a concise description of how GRα influences its specific functions: immune cells: these cells are primarily involved in the body’s immune response, including T cells, B cells, monocytes/macrophages, dendritic cells, and NK cells. GRα modulates their activity, such as cytokine production, cell proliferation, and differentiation, to maintain immune homeostasis and prevent excessive inflammation. Non-immune cells: these cells are not directly involved in the immune response but are essential for maintaining physiological balance. This category includes erythrocytes (red blood cells), platelets, endothelial progenitor cells, MSCs, and eosinophils. GRα influences processes such as oxygen transport, hemostasis, vascular repair, and tissue regeneration, as well as the modulation of allergic responses and inflammation in these cells. This table was generated with the assistance of AI using GPT-4. DC: dendritic cell; EPCs: endothelial progenitor cells; GRα: glucocorticoid receptor alpha; IFN-γ: interferon-gamma; MSCs: mesenchymal stem cells; NK: natural killer; TNF-α: tumor necrosis factor-alpha; Treg: regulatory T cell
The interaction between GRα and pro-inflammatory TFs, such as NF-κB and AP-1, is central to the stress response. Traditionally, pro-inflammatory and anti-inflammatory actions have been viewed as opposing forces in a “tug of war”. However, emerging evidence reveals a more nuanced relationship characterized by complementary co-regulation—dualism—between GRα and the NF-κB and AP-1 pathways throughout every phase of the disease process [26]. Rather than simply opposing each other, GRα and these pro-inflammatory TFs operate in a co-regulatory manner, dynamically adjusting gene expression according to the specific cellular context and inflammatory state (see below).
Building on the complex crosstalk between GRα and pro-inflammatory TFs, four seminal studies have uncovered a novel dual-phase modulation of chromatin accessibility by GRα and pro-inflammatory TFs [27–30]. In the initial phase of the host response (see below), GRα actively interacts with NF-κB or AP-1 to drive the transcription of pro-inflammatory genes, ensuring a strong immune response to external threats [27–29]. As homeostatic corrections advance, these interactions allow GRα to shift its role, aiding tissue recovery by promoting the transcription of anti-inflammatory genes [30]. During this phase, GRα modulates chromatin structure, shifting from promoting inflammation to restoring balance by activating anti-inflammatory pathways. This evolutionarily refined strategy illustrates how GRα, in coordination with pro-inflammatory TFs, ensures that the immune stress response is immediate and tightly regulated. By facilitating a rapid inflammatory reaction to threats while simultaneously preparing for anti-inflammatory and tissue-repair processes, GRα significantly contributes to efficient immune regulation and recovery during stress.
Temporal phases of GRα function and micronutrient’s impact on its regulation
The regulation of GRα involves six sequential phases (Table S1), each critical to its roles in stress response, immune modulation, and homeostatic corrections. Various factors, including hormone availability, chromatin accessibility, post-translational modifications, oxidative stress, and cellular signaling interactions, influence these phases [18]. Each phase is supported by specific micronutrients that facilitate GRα’s function and protect it from damage, ensuring effective cellular responses during stress and recovery (Table S2).
The general adaptation syndrome
While the discovery of GCs in 1950 [14] laid the groundwork for understanding their role in stress and inflammation, Selye [13] revolutionized the field in the 1930s and 1940s by systematically investigating the body’s response to stress. Through his pioneering work, Selye introduced the concept of general adaptation syndrome (GAS), illustrating how organisms respond to various stressors—physical, emotional, or environmental—predictably and stereotypically. He proposed that the body progresses through three distinct phases in response to stress: the Alarm Phase, characterized by the activation of immediate defense mechanisms; the Resistance Phase, in which the body adapts to the ongoing stressor; and the Exhaustion Phase, when the body’s resources become depleted. His research demonstrated that animals subjected to prolonged, multifactorial stress exhibited consistent physical changes, most notably adrenal cortex enlargement—an adaptation to increase GC production.
Selye later established that the failure of the HPA axis—the critical neuroendocrine system responsible for regulating the stress response—was pivotal in the body’s inability to cope with chronic or overwhelming stress. During the Exhaustion phase, the adrenal cortex’s capacity to produce cortisol becomes compromised, leading to the breakdown of homeostatic control, multi-system dysfunction, and immune dysregulation. This marks a shift from adaptive to maladaptive responses, characterized by dysregulated systemic inflammation and progression toward organ failure [13]. Given this understanding, therapeutic interventions such as intramuscular ACTH (adrenocorticotropic hormone) were explored [16] before the introduction of corticosteroids, aiming to stimulate cortisol production and mitigate immune dysregulation in patients with sepsis.
The general adaptation syndrome in critical illness
In critical illness, which represents the most extreme manifestation of the GAS, homeostatic corrections are essential for survival and require significant bioenergetic and metabolic resources. By the time patients reach life-support stages—such as needing vasopressors or mechanical ventilation—they are often nearing, or have reached, a state of complete exhaustion in their neuroendocrine compensatory mechanisms, cellular bioenergetic and metabolic reserves, and vital micronutrient stores [2]. This profound depletion greatly impairs GRα’s ability to effectively carry out the Priming Phase, similar to Selye’s Alarm Phase. This impairment diminishes GRα’s regulatory control over immune responses, reduces its support for essential organ functions, compromises anti-inflammatory mechanisms, and obstructs tissue repair—leading to a maladaptive response. Consequently, the body’s cumulative physiological burden, termed allostatic load [31], hinders recovery potential and significantly increases the risk of acute and long-term morbidity and mortality [1].
Understanding the evolutionary development of homeostatic correction mechanisms establishes the groundwork for appreciating the complex pathobiology underlying critical illness (Table 1). The GC-GRα system orchestrates targeted adjustments throughout critical illness, dynamically integrating signals from other hormonal and molecular pathways to maintain balance [1, 18, 32]. This adaptive capability allows the body’s responses to be precisely tailored to the specific context and target cells, enabling finely tuned adjustments that meet the unique demands of various tissues under both physiological and pathological conditions.
Central to recovery, the GC-GRα system regulates several key processes: immune modulation, which includes the regulation of inflammatory responses and immune cell activity; maintaining vascular and neural integrity through endothelial preservation and neurotransmitter modulation; energy control via mitochondrial function; the stress response and metabolism, which affect glucose and lipid homeostasis; balancing electrolytes through renal and mineralocorticoid interactions; and facilitating tissue repair through chromatin remodeling and cellular regeneration [1].
Vitamins and micronutrients play a crucial role in every phase of homeostatic corrections [2]. They are essential cofactors in metabolic processes, support GRα regulation (see supplementary information), enhance antioxidant defenses, and facilitate cellular repair. These functions are vital for maintaining physiological balance during stress and recovery. Increased metabolic demands and oxidative stress can quickly deplete these nutrients during severe illness. Throughout evolution, organisms have adapted to utilize these nutrients to boost mitochondrial energy production, regulate gene expression, and modulate immune responses. However, timely replenishment is essential to maintain resilience and promote recovery in the case of severe disease.
A previous publication initially outlined three major phases of homeostatic corrections in critical illness [33]. This review significantly updates that model (Figure 1). In an adaptive response, these phases progress successfully, leading to disease resolution and restoring homeostasis. In contrast, a maladaptive response prevents the completion of these phases, hindering recovery, perpetuating dysfunction, and potentially resulting in chronic disease or mortality.
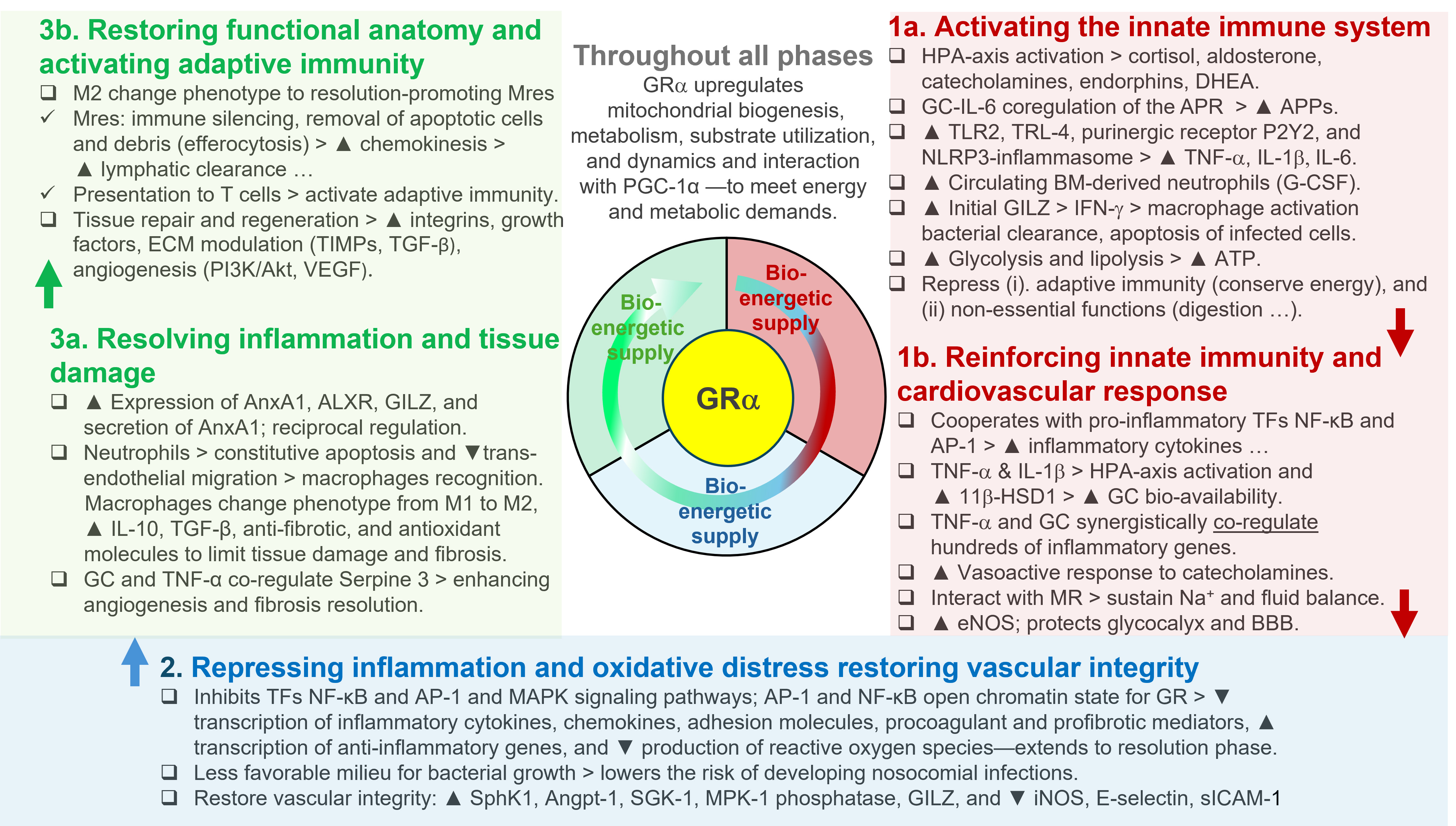
GRα regulation of the three major phases of homeostatic corrections. The GRα is a key regulator of vital physiological processes, playing a central role in sustaining life by coordinating the body’s adaptive responses to stress and maintaining a complex homeostatic balance. GRα serves two essential functions that are critical for survival: (i) ensuring an adequate bioenergetic supply by mobilizing glucose, fatty acids, and other substrates to meet the energy demands of cells and tissues under stress, and (ii) precisely regulating the neuroendocrine-immune axis, harmonizing interactions among hormones, neurotransmitters, and immune mediators. This finely tuned coordination allows GRα to modulate the body’s response across three distinct phases of adaptive homeostasis corrections: in the Priming Phase (1a and 1b), the GRα activates early cellular and molecular pathways that prepare the body for immediate defense, enhancing energy availability and initiating immune surveillance. During the Modulatory Phase (2), the GRα’s primary goal is to mitigate further damage by downregulating the inflammatory response and reducing oxidative stress. In the Restorative Phase (3a and 3b), GRα helps resolve inflammation and restore systemic balance. This phase promotes long-term stabilization by facilitating tissue repair, replenishing energy stores, activating adaptive immunity, and returning the neuroendocrine-immune system to its baseline state. By closely regulating these processes, GRα enables the body to adapt dynamically to environmental and internal challenges, protecting health and ensuring survival. It is important to recognize that disease processes can differ significantly based on specific conditions and individual factors. These phases may not always occur in a strictly linear manner, with varying degrees of overlap possible. Modified with permission from reference [33]. The arrows indicate progress to the next phase; the black triangle down ▼ = reduction; the black triangle up ▲ = increase; the open triangle > resulting effects. 11β-HSD1: 11beta-hydroxysteroid dehydrogenase type 1; ALXR: AnxA1 receptor; Angpt-1: angiopoietin-1; AnxA1: annexinA1; AP-1: activator protein 1; APPs: acute-phase proteins; APR: acute phase response; BBB: blood-brain barrier; BM: bone marrow; DHEA: dehydroepiandrosterone; ECM: extracellular matrix; eNOS: endothelial nitric oxide synthase; GC: glucocorticoid; G-CSF: granulocyte-colony stimulating factor; GILZ: glucocorticoid-induced leucine zipper; GR: glucocorticoid receptor; GRα: GR alpha; HPA: hypothalamic-pituitary-adrenal; IFN-γ: interferon-gamma; IL: interleukin; iNOS: inducible nitric oxide synthase; MAPK: mitogen-activated protein kinase; MKP-1: MAPK phosphatase 1; MR: mineralocorticoid receptor; Mres: resolution-promoting macrophages; Na+: sodium; NF-κB: nuclear factor-kappa B; NLRP3: nod-like receptor family pyrin domain containing 3; PGC-1α: peroxisome proliferator-activated receptor gamma coactivator-1 alpha; PI3K: phosphoinositide 3-kinase; SGK-1: serum glucocorticoid kinase-1; sICAM-1: soluble intercellular adhesion molecule-1; SphK1: sphingosine kinase 1; TFs: transcription factors; TGF-β: transforming growth factor beta; TIMPs: tissue inhibitors of metalloproteinases; TLR2: toll-like receptor 2; TLR4: toll-like receptor 4; TNF-α: tumor necrosis factor-alpha; VEGF: vascular endothelial growth factor
Priming Phase: activating innate immunity, bioenergetic supply, and cardiovascular response
Activating immunity and bioenergetic supply. Following the activation of the HPA axis, the GC-GRα signaling system plays a key role in enhancing the body’s initial immune response by ensuring energy availability, supporting cardiovascular function, and maintaining vascular integrity. This essential activation prepares the body to mount a rapid defense against various stressors, including pathogenic and inflammatory stimuli. GC-GRα is vital for activating and mobilizing key immune cells—such as T cells, B cells, natural killer (NK) cells, dendritic cells, monocytes/macrophages, and neutrophils—thereby regulating both innate (early) and adaptive (late) immune responses. Additionally, the GC-GRα complex enhances cellular energy capacity and efficiency by promoting mitochondrial biogenesis [34, 35], boosting glucose metabolism, and facilitating energy production through glycolysis and fatty acid breakdown [36]. Throughout all phases of homeostatic correction, the complex supports bioenergetic stability by modulating oxidative stress responses and preserving mitochondrial integrity [34, 35]. This sustained support is essential for meeting the metabolic demands of immune activation, tissue repair, and systemic recovery, as mitochondria play a crucial role in energy production, cell survival, and adaptive signaling [2].
Priming innate immunity through key mechanisms. Following the activation of innate immunity, the GC-GRα complex enhances innate immunity through several mechanisms. First, the GC-GRα complex initiates and modulates the systemic and hepatic acute-phase protein (APP) response via IL-6 and nitric oxide (NO), with GRα co-regulating alongside IL-6 [29, 37]. This response promotes the production of essential proteins, such as C-reactive protein (CRP) and serum amyloid A (SAA), which are crucial for amplifying inflammation and innate immunity. Second, the GC-GRα complex induces the expression of critical immune receptors, including toll-like receptors 2 and 4 (TLR2 and TLR4) [38], p38 mitogen-activated protein kinase (p38 MAPK), the NLR (nod-like receptor) family pyrin domain containing 3 (NLRP3) inflammasome [39, 40], and the purinergic receptor P2Y2R [41]. These receptors are vital in recognizing pathogen-associated molecular patterns (PAMPs) and danger-associated molecular patterns (DAMPs), enabling the innate immune system to respond quickly and effectively to infections and cellular stressors. Lastly, the collaboration of GR with pro-inflammatory TFs like NF-κB and AP-1, as well as TNF-α (tumor necrosis factor-alpha), regulates the transcription of hundreds of genes central to innate immunity (see below).
Amplifying early immune activation through cooperation with pro-inflammatory TFs. In addition to its role in priming innate immunity, the GC-GRα complex amplifies immune responses by collaborating with pro-inflammatory TFs such as NF-κB, AP-1, and TNF-α. This collaboration alters chromatin structure and regulates previously inaccessible genes [28, 30], enabling the induction of robust pro-inflammatory responses [27–29]. This synergy enhances essential immune functions, including leukocyte trafficking, chemokine production, and activation of complement components [29, 38]. Moreover, the GC-GRα complex facilitates the induction of NLRP3, sensitizing cells to extracellular ATP and triggering the release of pro-inflammatory molecules such as TNF-α, IL-1β, and IL-6 [39, 40]. Additionally, the GC-GRα signaling system operates in concert with other hormonal systems, including the HPA axis and thyroid hormones, to orchestrate a comprehensive and adaptive response that aligns with the body’s needs during critical stages of illness.
New understanding of the HPA axis response in critical illness. Initially, there is a brief surge in ACTH-driven cortisol production, followed by peripheral adaptations that maintain elevated cortisol levels without a high ongoing output. These adaptations include GC-GRα-induced modulation of hepatic gene expression, which reduces albumin and cortisol-binding proteins, decreases cortisol metabolism in the liver and kidneys, and leads to tissue-specific changes in GR expression and function. Such mechanisms ensure that cortisol availability and action are tailored to the body’s needs during critical illness [42].
Regulation of phagocytic activity and bacterial killing. The GC-GRα plays a crucial role in balancing immune responses by preventing excessive activation of phagocytic cells while simultaneously enhancing intracellular bacterial killing [43]. During the early phase of innate immunity, the upregulation of glucocorticoid-induced leucine zipper (GILZ) boosts interferon-gamma (IFN-γ) expression, promoting macrophage activation, bacterial clearance and the induction of apoptosis in infected cells. This dual action regulates the immune response by inhibiting pro-inflammatory TFs such as NF-κB and AP-1 while promoting anti-inflammatory mediators through GILZ [44–46]. This coordination ensures adequate pathogen clearance while preventing excessive inflammation.
Regulation in immune control. GC regulation is vital for maintaining immune control. Without adrenal GC production, pathogen clearance may occur more rapidly; however, this can result in increased mortality due to uncontrolled T-cell activation [47]. Pro-inflammatory cytokines enhance GC bioavailability by stimulating cortisol production and activating 11β-HSD1 (11beta-hydroxysteroid dehydrogenase type 1) [48, 49]. This synergy between cytokines and GCs strengthens early immune responses while preventing excessive phagocyte activation. During the Priming Phase, the GC-GRα complex also suppresses acquired immunity [11, 38, 47]. It restricts non-essential functions like digestion, reproduction, and growth, conserving energy for the immediate needs of the innate immune system.
Cardiovascular response and endothelial protection. The GC-GRα is essential for improving cardiovascular responses and protecting endothelial cells. It enhances the sensitivity of adrenergic receptors and upregulates beta-adrenergic receptor expression in cardiac and vascular smooth muscle cells [5, 50, 51]. This enhancement increases the responsiveness of these cells to catecholamines during stress [52]. Additionally, the GC-GRα complex stimulates the synthesis and release of catecholamines from the adrenal medulla, ensuring a sufficient supply of these hormones during stressful conditions. It also enhances NO production by upregulating endothelial nitric oxide synthase (eNOS), increasing NO levels that help maintain vascular tone, reduce vascular resistance, and prevent endothelial dysfunction [53–55]. Furthermore, the GC-GRα modulates sodium retention and fluid balance through interactions with mineralocorticoid receptors [56]. The GC-GRα complex supports robust cardiovascular function and sustains vascular integrity during immune and stress responses through these mechanisms.
Antioxidant defense and vascular health. In addition to its cardiovascular effects, the GC-GRα complex promotes the upregulation of antioxidant enzymes and protective factors in endothelial cells, which helps reduce oxidative stress [57, 58]. By boosting the antioxidant capacity of endothelial cells, these actions offer ongoing protection to vascular tissues during immune responses, thus supporting long-term vascular health.
Modulatory Phase: repressing inflammation, mitigating oxidative stress, and restoring vascular integrity
After the reinforcement and stabilization of the initial immune response, the body transitions into the Modulatory Phase. The primary goal during this phase is to prevent further damage by downregulating inflammation and reducing oxidative stress. This shift, directed by the GC-GRα signaling system, is critical since uncontrolled inflammation and oxidative stress can hinder recovery by damaging tissues and impairing GRα function. Equally important is restoring vascular integrity, as adequate blood flow and organ perfusion are essential for tissue repair and preventing further deterioration.
Balancing inflammation and repair. The GC-GRα system plays a crucial role in balancing inflammation and repair. Finely tuning the inflammatory response decreases the immediate risk of tissue damage and minimizes the likelihood of chronic inflammation that could hinder recovery. Simultaneously, the system begins restoring vascular integrity, ensuring that nutrient delivery and blood flow to affected tissues are sustained or improved. This phase requires a careful balance between suppressing harmful inflammatory and oxidative processes and promoting early tissue repair and recovery, laying a foundation for the subsequent healing phase.
Anti-inflammatory mechanisms of the GC-GRα complex. During this phase, the GC-GRα complex exerts powerful anti-inflammatory effects by inhibiting key inflammatory pathways, including NF-κB, AP-1, and MAPK [59]. Simultaneously, it enhances the transcription of anti-inflammatory genes and the NF-κB inhibitory protein IκBα (IkappaBalpha) [10, 25], further strengthening the anti-inflammatory response. The interaction between GCs and NF-κB is stimulus-specific, allowing for a flexible and adaptive mechanism to regulate inflammation effectively [30].
Collaboration with pro-inflammatory TFs. A notable feature of this phase is the cooperative interaction between the GC-GRα complex and pro-inflammatory TFs, which paradoxically enhances the anti-inflammatory response. This collaboration supports the resolution of inflammation and the stabilization of vascular function [30]. Chromatin accessibility is crucial in this process, enabling the GC-GRα complex to collaborate with pro-inflammatory TFs, thereby amplifying GC’s anti-inflammatory effects. For instance, AP-1 primes chromatin, facilitating the binding of the GC-GRα complex to suppress inflammation, while increased chromatin accessibility allows the GC-GRα complex to fine-tune NF-κB activity [28].
Preventing nosocomial infections in critical illness. In critical conditions such as acute respiratory distress syndrome (ARDS) and sepsis, dysregulated inflammation impairs neutrophil function, increasing the risk of secondary infections [60–63]. Effectively managing inflammation through a strong anti-inflammatory response significantly lowers the risk of nosocomial infections [64–67]. In survivors of ARDS, reduced levels of inflammatory cytokines are linked to inhibited bacterial growth, while in non-survivors, elevated cytokine levels promote pathogen proliferation [68]. Prolonged GC treatment reduces systemic and pulmonary inflammation, decreasing the infection risk by shortening mechanical ventilation duration, limiting the potential for pathogen growth, and enhancing neutrophil function [43, 68–71].
Restoring vascular integrity through endothelial regulation. In addition to controlling inflammation, the GC-GRα complex is essential for restoring vascular integrity by upregulating mediators that support endothelial cell homeostasis. It promotes sphingosine kinase 1 (SphK1), which produces sphingosine-1-phosphate (S1P), a vital molecule for maintaining endothelial barrier function. Furthermore, the complex enhances angiopoietin-1 (Angpt-1) expression, stabilizing endothelial cell junctions and reducing vascular permeability. These actions are critical for ensuring vascular stability during the recovery process.
Supporting vascular stability and endothelial health. The GC-GRα complex induces MAPK phosphatase-1 (MKP-1) [72], which reduces inflammation by inactivating MAPKs and upregulates serum glucocorticoid kinase-1 (SGK-1) [73], promoting cell survival and supporting endothelial stability. Additionally, GILZ, another important mediator upregulated by the complex [74], inhibits pro-inflammatory TFs such as NF-κB, further safeguarding vascular integrity. The GC-GRα complex enhances NO production through eNOS [53–55], maintaining vascular tone, reducing vascular resistance, and preventing endothelial dysfunction. Collectively, these actions help preserve the vascular system as the body transitions into the resolution phase of homeostatic corrections.
Experimental evidence in sepsis. In experimental sepsis, low-dose GC treatments, such as hydrocortisone or dexamethasone, have been shown to preserve the endothelial glycocalyx, maintain the vascular barrier, reduce interstitial edema [75, 76], and enhance mesenteric blood flow—each contributing to the resolution of organ injury [77]. These findings underscore the critical role of the GC-GRα signaling system in facilitating the transition from inflammation to tissue repair and recovery. They emphasize the importance of the ongoing modulation of this pathway to support long-term recovery in critically ill patients.
Restorative Phase: resolving inflammation, facilitating tissue repair, restoring normal structure, and activating adaptive immunity
The sequential phases of inflammation represent an adaptive response when systemic inflammation is properly regulated. During the Restorative Phase, the GC-GRα complex plays a crucial role in resolving inflammation, aiding tissue repair, restoring normal tissue architecture, and activating adaptive immunity. In contrast, maladaptive responses emerge when the GC-GRα complex fails to downregulate inflammation adequately and does not promote tissue repair, leading to incomplete recovery. This dysfunction can result in persistent low-grade systemic inflammation, impaired tissue regeneration, and chronic inflammatory conditions, ultimately prolonging recovery and contributing to long-term organ damage dysfunction [1].
Timely downregulation of inflammation. Disease resolution necessitates the prompt downregulation of systemic inflammation during the Modulatory Phase. In the early stages of critical illness, uncontrolled inflammation can lead to prolonged organ support, depletion of neuroendocrine compensation, cellular bioenergetic reserves, and essential micronutrients—all vital for an adaptive response. Persistent inflammation, driven by NF-κB, worsens oxidative stress and micronutrient depletion. When GRα-mediated downregulation is inadequate, patients may experience slow improvement (partial failure) or no improvement (complete failure). This insufficient response continues to cause tissue damage, increasing the risk of in-hospital mortality or chronic morbidity among survivors [78]
From pro-inflammatory to pro-resolving mediators. During the Restorative Phase, resolving inflammation involves an active process marked by a transition from pro-inflammatory to pro-resolving mediators. As the inflammation subsides, the activated GC-GRα complex orchestrates several pro-resolution mechanisms, affecting the phenotypes of immune cells like granulocytes and macrophages. The GC-GRα complex enhances the expression of vital pro-resolving proteins, including AnxA1 (annexinA1), its AnxA1 receptor (ALXR), and GILZ through genomic mechanisms. These molecules play an essential role in regulating the resolution phase of inflammation, particularly in macrophages and neutrophils.
Clearance of cellular debris and preparation for tissue repair. When inflammation starts, damaged tissues release debris and intracellular molecules due to cell injury or death, signaling immune cells to begin healing [79]. Effective tissue repair relies on the timely removal of this debris [79]. During this phase, the GC-GRα complex facilitates the clearance of damaged cells through phagocytosis [57, 80] and aids in the breakdown of fibrin and clots, creating a clean foundation for tissue repair.
Supporting cellular regeneration and structural restoration. The GC-GRα complex is crucial for cellular regeneration and structural repair, assuming various roles in tissue healing and recovery. It stimulates the production of growth factors, including vascular endothelial growth factor (VEGF), fibroblast growth factor (FGF), and transforming growth factor-beta (TGF-β), which encourage cell proliferation, angiogenesis, and tissue regeneration. Moreover, the complex boosts the synthesis of extracellular matrix (ECM) components, such as collagens, elastin, and fibronectin, which are vital for restoring tissue architecture and ensuring structural integrity support [1].
Restoration of anatomical structure. The primary goal of this phase is to restore the physiological anatomical structure and functionality. The GC-GRα complex is crucial in directing tissue remodeling, ensuring that affected organs and systems recover their proper functionality [1]. This process involves the physical reconstruction of tissues and the normalization of cellular activities, ultimately restoring health and homeostasis. Effective tissue repair requires the local tissue environment to return to a homeostatic state with normalized pH, oxygen levels, and other biochemical conditions.
Granulocyte and macrophage transitions in inflammation resolution. In neutrophils, GILZ expression significantly increases in GC-treated ARDS patients [81], promoting their apoptosis and reducing their inflammatory potential. Neutrophils play a critical role by recognizing and clearing apoptotic cells through efferocytosis, enabling their transition from a pro-inflammatory (M1) to an anti-inflammatory (M2) phenotype [82, 83]. M2 macrophages release anti-inflammatory mediators, enhance tissue regeneration, and support lymphatic clearance through improved chemokinesis. Simultaneously, the GC-GRα complex fine-tunes innate immune responses, contributing to effective inflammation resolution. See reference [1] for a detailed review.
Role of AnxA1 and macrophages in tissue repair. The GC-mediated AnxA1 peptide (Ac2–26), acting through the ALXR, is essential for clearing apoptotic cells during tissue repair [84]. In the later stages of repair, macrophages undergo a phenotypic transition to a resolution-promoting phenotype [Mres (resolution-promoting macrophages)], which minimizes tissue damage and fibrosis [1]. These Mres secrete antifibrotic and antioxidant molecules, further supporting tissue regeneration. Additionally, GCs affect gene expression in monocytes, activating anti-inflammatory pathways that facilitate inflammation resolution and promote tissue repair [57, 85].
GC-GRα and TNF-α co-regulation in tissue remodeling. The GC-GRα complex, in synergy with TNF-α, co-regulates the expression of SERPINE1 (plasminogen activator inhibitor-1), a key mediator of tissue remodeling. SERPINE1 is integral to ECM dynamics, angiogenesis, and fibrosis. During inflammation, TNF-α upregulates SERPINE1, promoting ECM accumulation. In the resolution phase, GCs modulate SERPINE1 expression, striking a balance between pro-fibrotic and pro-resolving pathways. This regulation enhances angiogenesis and promotes fibrosis resolution, facilitating effective tissue repair and restoration [29, 39, 40].
Preserving muscle mass during prolonged illness. During extended illness, immobility and systemic catabolic stress frequently result in considerable muscle loss. GCs are vital in preserving muscle mass and function by regulating protein metabolism and decreasing muscle catabolism. This protective effect prevents severe muscle wasting, which is essential for recovery and effective rehabilitation [86].
Finally, the GC-GRα complex plays a crucial role in coordinating the body’s transition from acute stress responses to long-term homeostatic balance restoration, helping to safeguard against the potentially harmful effects of chronic or repeated stress.
Conclusions
The GC-GRα regulatory network, developed over 450 million years, is essential for maintaining and restoring homeostasis, especially during critical illness. Its ability to precisely modulate immune responses, protect vital tissues, and promote cellular recovery underscores its essential role in immediate defense and long-term healing. The GC-GR system has been uniquely adapted to meet the complex demands of homeostasis under the stress of critical illness, highlighting the importance of supporting its function as a therapeutic priority. Recent reviews have further emphasized other significant factors influencing the response to GC therapy [3, 31].
Additionally, the effectiveness of the GC-GRα complex relies closely on essential micronutrients, which are often significantly depleted in critically ill patients. This highlights the urgent need to reassess current treatment strategies to include nutrient replenishment, thereby maximizing the efficacy of GC interventions. Refer to the latest European Society for Clinical Nutrition and Metabolism guidelines for micronutrient supplementation in critically ill patients [87]. Adjusting GR activity during the phases of homeostatic corrections is crucial for developing innovative therapeutic approaches aimed at mitigating pathological pathways and restoring homeostasis. Integrating a deeper understanding of the GC-GRα network into clinical practice can transform therapeutic strategies, paving the way for improved outcomes and restoring homeostasis for critically ill patients.
Abbreviations
ACTH: | adrenocorticotropic hormone |
ALXR: | annexinA1 receptor |
AnxA1: | annexinA1 |
AP-1: | activator protein 1 |
ARDS: | acute respiratory distress syndrome |
ECM: | extracellular matrix |
eNOS: | endothelial nitric oxide synthase |
GAS: | general adaptation syndrome |
GC: | glucocorticoid |
GR: | glucocorticoid receptor |
GRα: | glucocorticoid receptor alpha |
HPA: | hypothalamic-pituitary-adrenal |
MAPK: | mitogen-activated protein kinase |
Mres: | resolution-promoting macrophages |
NF-κB: | nuclear factor-kappa B |
NLRP3: | nod-like receptor family pyrin domain containing 3 |
NO: | nitric oxide |
SERPINE1: | plasminogen activator inhibitor-1 |
TFs: | transcription factors |
TNF-α: | tumor necrosis factor-alpha |
Supplementary materials
The supplementary tables for this article are available at: https://www.explorationpub.com/uploads/Article/file/101426_sup_1.pdf.
Declarations
Acknowledgments
This work was previously published as a preprint. DOI: 10.20944/preprints202409.1823.v1. During the preparation of this work, the author used ChatGPT-4 to generate tables and Consensus and Google Scholar for the literature search. The author acknowledges the assistance of AI-powered tools (GPT-4) in the table’s (Table 1, 2, and 3) research, structuring, and refinement. The author reviewed and edited the content as needed and takes full responsibility for the content of the publication.
Author contributions
GUM: Conceptualization, Investigation, Writing—original draft, Writing—review & editing.
Conflicts of interest
The author declares that there are no conflicts of interest.
Ethical approval
Not applicable.
Consent to participate
Not applicable.
Consent to publication
Not applicable.
Availability of data and materials
Not applicable.
Funding
Not applicable.
Copyright
© The Author(s) 2025.
Publisher’s note
Open Exploration maintains a neutral stance on jurisdictional claims in published institutional affiliations and maps. All opinions expressed in this article are the personal views of the author(s) and do not represent the stance of the editorial team or the publisher.