Abstract
The on-site, rapid, and intelligence detection methods are the wave in food safety. Recently, intelligent point-of-care test (iPOCT) methods serve as a promising alternative for advanced monitoring in food safety. By integrating smartphones with various detection methods, iPOCT methods demonstrate unique merits. Compared with lab-dependent instruments, iPOCT strategies have a short turnaround time (several minutes), high accuracy (μm level or less), and portability (smartphones). This work discussed principles of optical and electrical iPOCT methods, including absorbing light, fluorescence, chemiluminescence, potentiometry, voltammetry, impedance spectroscopy, and amperometry. The review emphasizes the practical applications for testing chemical and biological hazards in complex food matrices. The commercialization, challenges, and future trends of iPOCT are discussed as well.
Keywords
Food safety, hazardous factors, intelligent point-of-care test, commercialization, opportunitiesIntroduction
Today, people have increasingly higher demands for food quantity and quality. Food safety threatens life and health and requires advanced intelligent detection technology to prevent contaminated food and raw materials from entering the food chain. The main hazardous factors in food are biological, chemical, and physical [1]. Biological hazardous factors include bacteria, fungi, and viruses. The most common bacteria are Salmonella [2], Escherichia coli [3] Vibrio parahaemolyticus [4], Enterobacter sakazakii [5], Listeria monocytogenes [6] and Clostridium perfringens [7]. Human food consumption containing foodborne bacteria can result in kidney failure, concurrent inflammation (meningitis, gastroenteritis), and even death [8]. Fungal toxins commonly detected in food include aflatoxins [9], fumonisins [10], ochratoxin A (OTA) [11], deoxynivalenol [12], and zearalenone (ZEN) [11], which can cause cancer, teratogenicity, mutagenicity, acute poisoning, and chronic poisoning [13]. Among foodborne viruses, norovirus [14], hepatitis virus [15], and Norwalk-like viruses (NLVs) [16] frequently harm human health. They have strong infectivity and fast transmission speed and are transmitted through contact between people or by consuming contaminated food and water. Mild symptoms include vomiting and diarrhea, while severe cases can lead to death [17], especially among older adults, people with weakened immunity, and newborns [18]. Chemical hazardous factors include heavy metal ions [19], pesticides [20], additives [21], toxins [22], and antibiotics [23]. Heavy metals present in food mainly include mercury [24], lead [25], chromium [19], copper [26], and manganese [27]. They primarily cause harm to the nervous system, immune system, liver, digestive system, cancer, and even genetic problems [28]. Pesticides mainly include organophosphorus [29], carbamate [30], organochlorine [31], and neonicotinoid [32]. Residual pesticides can easily cause acute poisoning and death. Reported food additives include ascorbic acid [33], nitrite [34], and beet red [35], which adversely affect the gastrointestinal tract, skin, respiratory system, nervous system [36]. Reported antibiotics include quinolones [37], tetracyclines [38], and amoxicillin [39]. Antibiotics abuse caused the emergence of “superbugs”, increasing bacterial resistance, and making disease treatment more difficult [40]. Physical hazardous factors such as particulate matter and stones entering food are not related to intelligent detection, so this review does not provide a detailed introduction on them.
To prevent food contaminants from entering the food chain, international organizations and governments worldwide have established strict limit standards. Therefore, advanced high-sensitivity intelligent detection technology is urgently needed. Currently, methods for detecting contaminants in food include chromatography [41], chromatography-mass spectrometry [42], and immunological methods [43]. Chromatography separates pollutants based on molecular weight, while mass spectrometry has high selectivity based on different pollutant ion pairs. The above methods have high accuracy and reliability, but their shortcomings are that the equipment is expensive and requires professional technical personnel [44]. Compared with large or expensive desktop instruments, the intelligent point-of-care test (iPOCT) is simple and easy to use. Combining multiple disciplines, such as immunology nanomaterials, and minimization, iPOCT methods can obtain low detection limit, high specificity, wide linear range, and satisfied recovery rate. The antibody-antigen recognition improves the robustness and specificity of iPOCT in practical applications [45]. Nanomaterials with large specific surface areas achieve a high loading capacity and enhanced electrochemical signal transferring for signal amplification. The interdisciplinarity can enhance the development of advanced iPOCT for special applications in diversified food samples. Therefore, iPOCT methods are ideal for on-site, sensitivity detection in food safety.
The rapid development of smartphones provides unprecedented opportunities for the intelligentization of immunoassays, overcoming the problems of real-time feedback, the inability to upload big data, and the inability to provide intelligent supervision methods. Currently, there are 3.5 billion smartphone users worldwide. In the intelligent application scene of big data and cloud computing, intelligent detection has moved from theoretical research to practical applications, playing an increasingly important role in food safety [46]. The intelligent detection mode has advantages such as low cost, portability, user-friendliness, and ease of operation. IPOCT fully utilizes high-definition pixels, light emitting diode (LED) light sources, and wireless Bluetooth network connections built into smartphones. Its advantages include accurate and efficient performance, diverse modules, flexible use, easy operation, real-time data uploading and sharing, and a user-friendly interface. It is suitable for on-site detection of food contaminants [47]. Specifically, iPOCT reduces human errors by reducing manual operations, integrating multiple platforms such as absorbing light, fluorescence, microscopy, electrochemistry, and providing various testing modes suitable for different substrates and application scenarios.
With the development of intelligent detection technology, there are already good reviews, but most of them focus on medical detection and intelligent environmental detection. There are a few technical comments on commercial iPOCT products for food safety. This article reviews intelligent detection in food, including intelligent optical and electrical detection, and analyzes their advantages and disadvantages (Table 1). Secondly, we analyze the commercial trends of intelligent detection in food safety applications and propose challenges and opportunities. This article provides interdisciplinary knowledge for food safety, analytical chemistry, medical testing, instrumentation, materials science, immunology, etc., and provides a theoretical basis for the application of intelligent detection.
Advantages and disadvantages of iPOCT
iPOCT | Methods | Advantage | Disadvantage |
---|---|---|---|
Light iPOCT | Absorb light | Results by naked eye, microvolume, high-throughput, template diversification, flexibility | Low sensitivity |
Fluorescence | High sensitivity, multiple probe materials, biocompatibility, short time | Instability, toxic label | |
Chemiluminescence | High sensitivity, waving external light source | Environmental influences/instability | |
Electrochemical iPOCT | Potentiometry | Easy and fast operation, high accuracy and precision | Long analysis time |
Voltammetry | High accuracy and good reproducibility, wide range of application | Current interference, noise current | |
Impedance spectroscopy | Low system interference, simple data analysis | Uncertain data interpretation | |
Amperometry | Large analysis range, high sensitivity | Interference result, short life |
Optical iPOCT
Optical detection uses optical modules’ absorption or emission characteristics as output signals for qualitative or quantitative measurements [48]. Each analyte responds differently to light, producing a unique response pattern. Optical iPOCT includes absorbing light, fluorescence, and luminescence detection methods. It has been used to detect antibiotics [49], amino acids [50], iodine in food salt [51], vitamins [52], water content [53], metal ions [54], fungal toxins [55], pesticides [56], interferon [57], food freshness [58] and chiral mixtures [59].
Absorbing Light iPOCT
Absorbing light iPOCT is widely used in immunological detection strategies. Transforming color into ideal light signals is a crucial breakthrough point. It includes lateral flow absorbing light iPOCT [60], microfluidic absorbing light iPOCT [61], and enzyme absorbing light iPOCT [62].
As signal sources, lateral flow absorbing light iPOCT uses absorbent dyes such as colloidal gold, latex microspheres (LM), etc. These labeled materials have low sensitivity and light signal amplification strategies such as gold clusters [63], gold rods [64], and magnetic bead enrichment [65] need to be introduced. Gold nanoparticles (AuNP) are currently the most widely used technology for qualitative and semi-quantitative detection due to their color-changing properties caused by the excellent aggregation and dispersion performance of AuNP in solution systems. The sensitivity of this method can reach the ng level [66]. However, it is rarely used for quantitative detection.
The advantage of microfluidic absorbing light iPOCT is that it only requires a µL-level sample size to achieve high-throughput detection of contaminants in food through multiple channels. The diversity of microfluidic chip materials is characteristic, such as glass [67], silicon [68], polymethyl methacrylate (PMMA) [69], polydimethylsiloxane (PDMS) [70], and polycarbonate (PC) [71]. Machado et al. [72] used standard soft lithography techniques to prepare microfluidic chips in PDMS. The simple procedure is to design the pattern in computer-aided diagnosis (CAD) software and transfer it to an aluminum-coated substrate, then use an aluminum etching machine to wet-chemical etch the aluminum pattern to make a silicon material capillary microfluidic chip [72]. The light signals of smartphones are combined and analyzed. Firstly, it is worth noting that the membrane thickness can control the fluid velocity and thus be used to characterize the stability and reliability of the chip [73]. Secondly, the advantage of microfluidic iPOCT is template diversification, which can design various channel structures and sizes according to different needs. Thirdly, it can match multiple detection modes, such as optical detectors, smartphones, and mass spectrometry.
Enzyme absorbing light iPOCT judges the results by enzyme inhibition or enzyme catalysis principles, such as acetylcholinesterase (AChE) hydrolyzing acetylthiocholine iodide (ATCh) to produce thiocholine. When AChE is present, the combination of metal nanoparticles and thiocholine causes the metal nanoparticles to aggregate, causing the solution to change color from red to blue. However, organophosphorus pesticides [74] and carbamates [30] can inhibit AChE hydrolysis, so the above process cannot be achieved, and the solution color does not change. Xanthine oxidase (XOD), an enzyme that catalyzes xanthine, decomposes into uric acid (UA) and hydrogen peroxide (H2O2) in the presence of oxygen, and H2O2 further oxidizes 3,3’,5,5’tetramethylbenzidine (TMB) into oxidized TMB under the catalysis of horseradish peroxidase (HRP), producing visible blue. They are combined with paper-based sensors for visual detection of fish freshness [75].
Absorbing light iPOCT has been used to detect heavy metals and amino acids. For example, Ling et al. [76] prepared colloidal AuNP (Figure 1A) to labeled materials for sensitively detecting mercury ions (Hg2+) in water. 1-(4-isothiocyanate phenyl) ethylenediamine N,N,N’,N’-tetraacetic acid (ITCBE) was used as a dual-functional chelating agent to prepare specific antibodies by combining Hg2+ with a protein carrier. Larger multi-branched gold nanoflowers (AuNF) (Figure 1B) synthesized with AuNP as gold seeds and LM can improve detection sensitivity. Compared with AuNP test strips, AuNF test strips obtained lower limit of detection (LOD; 0.44 ng/mL) when detecting Hg2+, which is 20 times that of the AuNP-based strip (8.92 ng/mL). The detection limit of LM strips was 0.49 ng/mL, 18 times that of AuNP (Figure 1C) [76]. Compared with assembling multiple AuNP into multiple particles to improve the sensitivity, Cai et al. [77], used platinum-modified AuNP (Au@PtNP). They labeled them as probes on antibodies to assemble test strips to detect trace tenuazonic acid (TA) in apple juice and tomato sauce. The LOD can be lower than 0.39 ng/mL.
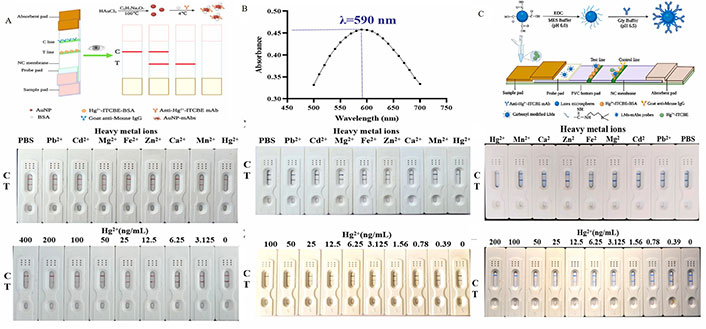
Establishment and characterization of strip test. A. Characterization of the AuNP-based strip (n = 3); B. characterization of the AuNF-based strip (n = 3); C. characterization of the LM-based strip (n = 3) [76]
Note. Reprinted from “Development of sensitive and portable immunosensors based on signal amplification probes for monitoring the mercury(II) ions,” by Ling S, Dong M, Xu Y, Xu A, Lin J, Lin M, et al. Biosens Bioelectron. 2022;217:114676 (https://www.sciencedirect.com/science/article/abs/pii/S0956566322007163). © 2022 Elsevier B.V.
Fluorescent iPOCT
Compared with absorbing light iPOCT, fluorescent iPOCT can directly or indirectly obtain signals from the mobile phone through signal amplification or non-amplification by optical signal transmission, which improves the detection sensitivity by one order of magnitude and enables high-sensitivity quantitative detection [78]. Currently, widely used fluorescent labeling materials include quantum dots [79], carbon-based nanomaterials [80], rare earth metal ions [81], and upconversion nanofluorescent materials [82]. Quantum dots are semiconductor fluorescent nanomaterial with advantages such as wide excitation range, controllable emission range, and high quantum yield [83]. The development of carbon-based nanomaterials overcomes the problem of the toxicity of quantum dots. The most reported carbon-based nanomaterials are carbon dots and graphene quantum dots, which have lower toxicity than quantum dots and good biocompatibility [84]. Rare earth-based nanomaterials are stable and bright fluorescent probes with high photobleaching and chemical degradation efficiency, significantly shortening the detection time (stokes displacement is 1–100 nm) [85]. The lanthanide chelate Eu3+ has high fluorescent intensity, photobleaching solid resistance, and long decay time (1,250 µs) [86], which delays the measurement time, eliminates natural fluorescent interference, and dramatically improves the sensitivity of the method [44]. Compared with traditional fluorescent labeling materials, upconversion nanoparticles have the advantages of high precision and accuracy in analysis. They have a large anti-stokes displacement, sharp emission peak, long life luminescence, and no photobleaching [87]. Because it is a unique material that can be excited by ultraviolet and emits visible light, it has the advantages of low fluorescent background and high signal-to-noise ratio [24].
Fluorescent iPOCT has been applied to detect toxins, pesticides, pollutants, antibiotics, and pathogenic bacteria markers intelligently. Hu et al. [88] developed a highly sensitive labyrinth-like quantum dot nanosphere (lqdb) (Figure 2). They combined it with a smartphone readout to achieve high-throughput rapid detection of aflatoxin B1 (AFB1) and ZEN in food. The lqdb coated with a thin silicon layer has a rough surface that achieves high antibody loading. The nanosphere has high colloidal stability and the advantage of easy functionalization. Signal changes of lqdb as the fluorescent label for dual-target iPOCT, combined with competitive immunoassay on lateral flow test strips, the results can be evaluated within 10 min. The detection limits of AFB1 and ZEN in agricultural products are 0.002 ng/mL and 0.02 ng/mL, respectively [88]. The detection time of this method is 20 min faster than other literatures [89]. Yang et al. [90] combined multicolor upconversion nanoparticle barcode technology with fluorescent image processing to develop a platform for quantitatively detecting fungal toxins. The design of multicolor upconversion nanoparticle-encoded microspheres can simultaneously detect different fungal toxins [90]. Zhang et al. [91] established a measuring stream test paper strip based on Eu3+ rare earth metal ions for determining vegetable cypermethrin residues. The innovative point of this method is the use of biomimetic material molecularly imprinted polymers instead of antibodies, which solves the problem of antibody variability in extreme environments. This method uses intelligent portable devices to obtain test results in 15 min and is suitable for rapid on-site testing [91]. Peng et al. [92] developed a “shutdown” carbon quantum dots fluorescent probe for detecting nitrite in food and water samples. Smartphones capture the changes in sample fluorescent, and different analytical performances under different detection modes are obtained and compared [92]. Wang et al. [37] reported a proportional fluorescent detection strategy constructed by three-doped graphene quantum dots for detecting ofloxacin (OFL) and its l-isomer levofloxacin (LEV) in milk. The detection limits of targets are as low as 46/67 nmol/L. With smartphones, OFL and LEV can be visually and quantitatively detected through RGB values output [37]. Yuan et al. [93] designed a proportional fluorescent strategy coupled with graphene nitride (g-C3N4) and Eu3+ to detect anthrax biomarker dipicolinic acid (DPA). As the concentration of DPA increases, the fluorescent of Eu3+ is significantly enhanced due to the antenna effect. The linear correction range is 0.1–15 µmol/L, and the detection limit is 13 nmol/L. Combined with smartphones, colorimetric and visual in-situ detection of DPA was performed [93].
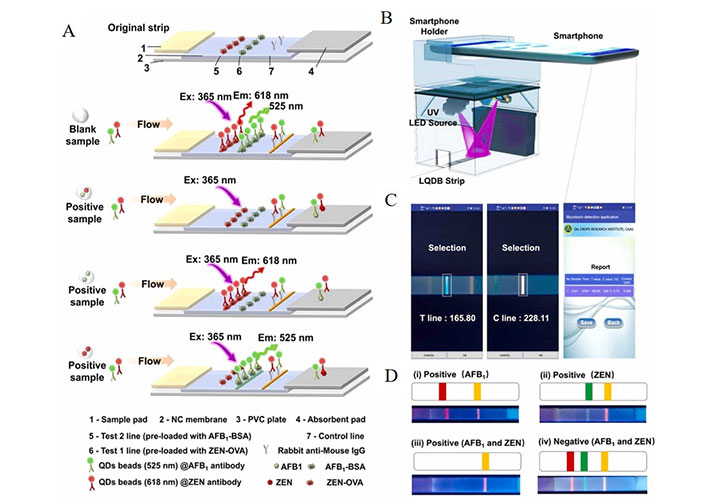
The AFB1 (or ZEN) mAb was labeled with the LQDB as a highly sensitive fluorescent signal compared with the Au labels. A. Schematic illustration of the principles of detection AFB1 and ZEN by the LQDB strip; B. integrated smartphone enables iPOCT platform diagram of the hardware; C. main contact surfaces of the detection app and sensing process; D. determination of AFB1 and ZEN by the LQDB strip [88]
Note. Reprinted from “Color-selective labyrinth-like quantum dot nanobeads enable point-of-care dual assay of Mycotoxins,” by Hu X, Huang L, Wang S, Ahmed R, Li P, Demirci U, et al. Sens Actuators B Chem. 2023;376:132956 (https://www.sciencedirect.com/science/article/abs/pii/S0925400522015994). © 2022 Elsevier B.V.
Chemiluminescence iPOCT
Luminescence is a type of non-thermal radiation emission from matter. Chemical luminescence is a phenomenon of light emission caused by chemical reactions, mainly oxidation reactions that release energy that can excite electrons. It has the advantages of high sensitivity, rapidity, large linear range, and not requiring an external light source. The substance that participates in energy transfer and releases emitting photons to generate energy in chemiluminescence reactions is called a chemiluminescent (CL) substrate, which has a high quantum yield, can form stable conjugates with antigens and antibodies, matches the properties of the labeled substance, and no toxic effect on organisms within the scope of use. CL substances can be divided into direct CL substances and enzyme-catalyzed reaction CL substances. Direct luminescent substances participate directly in luminescence immunoassays without the involvement of enzymes. Competing methods are mainly used for small-molecule detection, while sandwich methods are primarily used for macromolecular detection. Direct luminescent substances have unique luminescent groups that can directly label antigens and antibodies [94]. Luminescent substances rapidly emit light under NaOH and H2O2, such as luminol and tris(2,2’-bipyridine)ruthenium. Luminol emits 470 nm light in the NaOH and H2O2 system and has a high luminescence efficiency. The luminescent species produced in the excited state is methylamino phthalate copper [95]. Tris (2,2’-bipyridine)ruthenium is an electrochemiluminescence reagent that uses tripropylamine (TPA) as an electron donor and undergoes a specific chemical luminescence reaction due to electron transfer in an electric field. The process includes electrochemical and chemical luminescence processes, and an oxidation reaction occurs by losing electrons during luminescence [96]. Enzyme-catalyzed reaction luminescent substances make substrates luminesce by catalyzing enzymes, for example, alkaline phosphatase (ALP) and HRP. Luminol and its derivatives are decomposed into water and singlet oxygen through H2O2 under the catalysis of enzymes or metal compounds and then emit blue fluorescent [97]. The luminescence system of ALP and 1,2-dioxocyclohexane derivative (AMPPD) has a fast reaction rate and can obtain reliable results quickly. AMPPD contains 1,4-dioxane and phosphate groups. The former can connect the benzene ring and adamantane for cleavage and emission of photons, while the latter can maintain molecular structure stability [98].
Chemiluminescence iPOCT has been used to detect total antioxidant capacity (TAC), mycotoxins, and pesticides in food. Zhang et al. [99] utilized the excellent peroxidase activity of gold-doped copper hexacyanoferrate nanoparticles (Au@Cu-HCF), which can compete or reduce the substrate mechanism with food matrixes under the interaction of OH-free radicals, and undergoes chemiluminescence intensity changes based on concentration changes to explore the TAC of plant-origin food samples. They designed a sensor based on smartphones, effectively improving the TAC detection efficiency, and the lowest detection limit is 1 mmol/L [99]. Zangheri et al. [100] combined chemiluminescence with lateral flow immunoassay (LFIA) to establish a smartphone-based biosensor for quantitatively determining OTA in wine and instant coffee, with the lowest detection limit of 0.1 mg/L. This method can be easily completed without professional personnel. To amplify the signal, Ma et al. [9] used the dual-mode method of labeled and unlabeled double-stranded affinity to take advantage of the signal amplification characteristics mediated by double-stranded streptavidin. They combined chemiluminescence with smartphones to detect AFB1 in corn and peanuts, with a detection limit of 0.04 ng/mL, 1–5 orders of magnitude higher than single-channel detection [9]. The time required for one detection is no less than 30 min. To achieve a shorter detection time, Montali et al. [101] established a CL foldable paper biosensor for detecting AChE inhibitors. They measured H2O2 through luminescent substrates to determine the residual situation of organophosphorus pesticides in food matrixes. This method can be rapidly detected using 3D printing equipment connected to smartphones and requires only 25 min [101].
Electrochemical iPOCT
Electrochemical-based iPOCT is a type of on-site testing device for non-professionals, which has the advantages of portability, reliability, sensitivity, and small sample volume. It is widely used in rapid food analysis on-site. By measuring the electrical signals generated by changes in charge of the system, connecting with on-site intelligent devices, and using wireless communication technology such as built-in Bluetooth and hotspot signals to record, analyze and display the results of the electrical signals. The device is very simple, and it only needs to connect the circuit board and electrode and open the corresponding application software of the intelligent device to connect the communication structure to achieve electrochemical performance. The selection of electrodes is a vital link. For example, screen printing electrodes are one of the most common [102]. Electrochemical-based iPOCT detection methods include potentiometry [103], voltammetry [104], impedance [105], and amperometry [106].
Potentiometric iPOCT
Potentiometric measurement obtains results by monitoring the charge accumulation or potential change caused by the redox reaction on the electrode surface. Budetić and others [107] developed a direct potentiometric method for determining thiacloprid in orange peel, lemon peel, and banana peel based on the host-guest principle and advanced membrane science. By screening the concentrations of plasticizers and ion exchange electrode materials on the membrane, the electrode film with the highest response to the target substance was obtained [107]. Draz et al. [108] optimized the potential solid-state sensor by including a mesh membrane mixture containing 13 components. To improve the accuracy of detection, Gil et al. [109] combined a high-performance liquid chromatography (HPLC)-potential detector, separated the target substance by chromatography column, and connected the miniaturized amine-selective electrode to determine the results. Although this method effectively separates the target substance in the food sample before detection, it is not conducive to on-site iPOCT detection. To realize efficient and portable on-site detection, electrochemical devices are being researched for miniaturization. The entire equipment can be miniaturized when the microelectrochemistry analyzer is integrated with a portable potentiometer with a Bluetooth interface. It has the advantages of small sample size, portability, high automation, waste production below 4 mL, and microsystem life of no less than 4 months [110].
Potentiometric iPOCT has been widely used in pesticide residue and antibiotic detection. For example, Ge et al. [111] prepared a wireless intelligent portable device to determine terbutaline in meat samples. This technology uses graphene-like molybdenum disulfide, platinum-palladium nanoparticles, and carboxylated graphene to prepare layer-layer nanocomposite materials with high surface area and strong catalytic properties. It is connected with a portable intelligent phone potentiometer to achieve the lowest detectable limit of 0.18 μmol/L for terbutaline [111]. The laboratory used silver nanoparticle (AgNP) stabilized black phosphorus (BP)-modified halloysite nanotube (HNT), modified screen-printed carbon electrodes (SPCE) nano-materials combined with smartphones to perform potential measurements on maleic hydrazide in carrots, with detection limit of 0.3 μmol/L [112], which is higher than the detection limit in the previous literature.
Voltammetric iPOCT
Voltammetry obtains results by measuring the relationship between electrode potential and the current passing through an electrolytic cell obtained by resistance measurement. Since the formula is expressed as voltage compared to current, it is defined as voltammetry. The method measures the potential within a prescribed scan range, and the current signal is proportional to the target substance concentration. It has the advantages of accuracy, good precision, sensitivity, and selectivity [113]. The voltammetry methods used in food safety testing include square wave voltammetry (SWV), cyclic voltammetry (CV), and differential pulse voltammetry (DPV). For example, a multivariable analysis platform combining linear scanning, CV, and SWV was developed in conjunction with a smartphone system, successfully used for fingerprint identification of honey samples. Smartphones reduce costs while reducing the number of procedures for non-expert users to fit simulations. The platform allows measurements to be done in real-time at a distance, improving autonomy and portability [114]. Moreover, multiple heavy metals can be simultaneously qualified via a DPV POCT, providing LODs of 0.296 (Cd), 0.055 (Cu), 0.351 (Hg), and 0.025 (Pb) μmol/L in tap water [115]. Morawska et al. [116] established a SWV method to explore the influence factors such as electrolyte pH, composition, square wave parameters, and scanning rate. Nanomaterials have high specific surface area characteristics, making the electrode modified with nanomaterial have better signal quality [117]. For example, Nixon et al. [118] analyzed the electrochemical sensor designed using CV and DPV techniques. The LaNiO4-functionalized carbon nanofiber (f-CNF) modified electrode was used, which had high sensitivity (0.2899 µA·µmol/L–1·cm–2), low detection limit (LOD 6 nmol/L), and good response and recovery rate [118]. Yadav et al. [119] used molybdenum disulfide nanoparticles (nMoS2NPs) to modify coated glass for the electrochemical detection of ampicillin. The electrochemical platform has high sensitivity, a wide linear range, excellent stability, and reproducibility [119]. And voltammetry iPOCT has been widely used in heavy metal detection. For example, detecting heavy metal ions in liquid food can be evaluated using a gold/platinum electrode combined with a smartphone as a terminal signal receiver. The detection limit can reach as low as 2 μg/L [27].
Impedance spectroscopy iPOCT
Impedance spectroscopy iPOCT is an analytical method that explores the relationship between alternating current impedance frequency changes and alternating current signals in batteries under equilibrium or stable direct current conditions. The measurement results of this method are approximately linear conditions, making it easy to analyze and process, and it will not damage the battery during testing, making it an excellent non-destructive testing method. To improve electronic transfer efficiency, nanomaterials are used in the assembly components of potential iPOCT. Adding nanomaterials to electrode preparation can improve the catalytic and conductive properties of smart field iPOCT because nanoparticles increase the roughness of the electrode surface and enhance the electronic transfer efficiency, thereby enhancing the electrochemical signal. Metal-organic frameworks (MOFs) are highly sensitive, modifiable transduction elements with high specific surface area and adjustable porosity that can be designed and synthesized according to different metal particles and ligands. Impedance iPOCT has been reported in pesticide residue detection, such as Nagabooshanam et al. [120] who used a zinc-based metal-organic framework (MOF-Basolite Z1200) and AChE to modify a gold electrode and used electrochemical impedance spectroscopy (EIS) and other electrochemical techniques to detect organic phosphorus pesticides in food sensitively. The electrochemical microanalysis device (EμAD) has the advantage of a small sample size and requires only 2 μL of analyte. The detection limit can reach 6 ng/L, and the sensitivity is 0.598 μA/ng per active surface area (mm–2). It is worth mentioning that the reaction time for detecting dimethoate in actual vegetable samples is 50 s, with an incubation period of 20 s. Moreover, this device can be miniaturized with a commercially available inexpensive hand-held potentiometer connected via Bluetooth [120].
Amperometric iPOCT
Amperometric detection is the change of electrical signal caused by the reductant-oxidant (REDOX) reaction of the substance to be measured and then the concentration of the substance to be measured. Its principle of operation is to increase the voltage between the working electrode and the reference electrode to determine the generated current. With time, the amperometric i-t curve and the current changes during the redox process can measure the changing current. During this process, the electrode is fixed at a specific potential, which benefits electron transfer [121]. For example, Wu et al. [122] investigated the electrocatalytic behavior of glucose in alkaline media through density functional theory (DFT) calculations using metal-modified electrodes to adjust the catalyst’s electronic structure and surface properties. Shanmugam et al. [123] added ruthenium (Ru)-doped cobalt phosphide (R-CoP) to graphitic carbon nitride as an electrode modifier can increase the active surface area, enhance electron transferability, and reduce resistance. Electrochemistry can be evaluated by amperometric methods, which have good selectivity, repeatability, stability, and reproducibility. Notably, the developed method has better performance in terms of electrocatalytic detection than similar technologies [123].
Amperometric iPOCT is widely used in pesticide and biological toxin detection. For example, Caratelli et al. [124] developed an electrochemical platform based on office paper screen-printed electrodes and a filter paper pad loaded with enzymes and enzyme substrates (Figure 3A). It can detect oxy phosphonate, 2,4-dichlorophenoxyacetic acid, and glyphosate at the ppb level. Using a smartphone-assisted constant potential meter and measuring initial and residual enzymatic activity, the inhibition rate can be evaluated, proportional to the amount of sprayed pesticides. The instrument can detect 2,4-dichlorophenoxyacetic acid, glyphosate, and oxyphosphonates in the aerosol phase with detection limits of 30 ng/mL, 10 ng/mL, and 2 ng/mL, respectively [124]. Compared to an earlier antibody-free detection technology based on paper, the detection limit has increased by three orders of magnitude. Early work involved labeling synthetic peptides of natural substrates SNAP-25 with electroactive molecules methylene blue and fixing them to paper-based electrode. The electrode modified with AuNP (Figure 3B). Due to the botulinum neurotoxin serotype (BoNT) in orange juice labeled samples, the signal decreased directly to the BoNT. The smartphone-assisted potentiostat can detect BoNT, with linearity up to 1 nmol/L and a detection limit of 10 pmol/L [125].
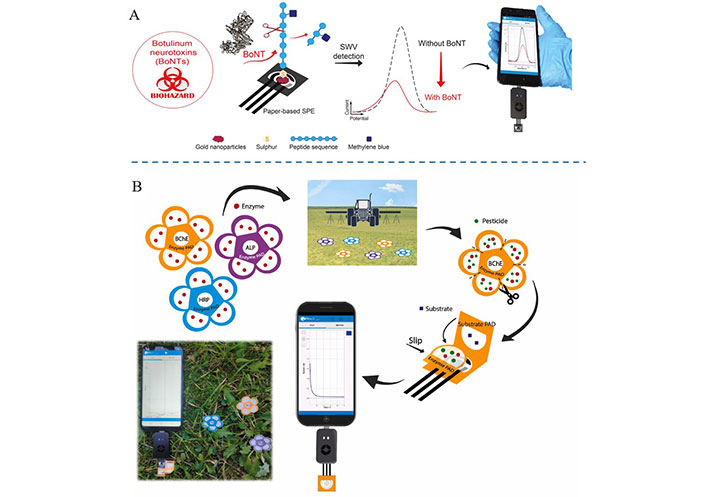
The pesticide and biological toxin ion were detected by amperometric iPOCT. A. The electrochemical platform based on office paper screen-printed electrodes combined with smartphone detect the BoNTs [125]; B. the flower-like origami paper-based electrochemical device able to detect pesticides using a smartphone-assisted potentiostat [124]
Note. Figure 3A is adapted from “Paper-based electrochemical peptide sensor for on-site detection of botulinum neurotoxin serotype A and C,” by Caratelli V, Fillo S, D’Amore N, Rossetto O, Pirazzini M, Moccia M, et al. Biosens Bioelectron. 2021;183:113210 (https://doi.org/10.1016/j.bios.2021.113210). © 2021 Elsevier B.V.; Figure 3B is reprinted from “A paper-based electrochemical device for the detection of pesticides in aerosol phase inspired by nature: a flower-like origami biosensor for precision agriculture,” by Caratelli V, Fegatelli G, Moscone D, Arduini F. Biosens Bioelectron. 2022;205:114119 (https://doi.org/10.1016/j.bios.2022.114119). © 2022 Elsevier B.V.
Commercialization
There have been many reports of smartphone-based laboratory tests for food safety, but few of these devices have made it into commercial factory products. IPOCT, which has been commercialized, is mainly a side-flow product. For example, RIDA®QUICK, a test strip product for mycotoxin detection based on mobile phone photography, uses water and organic phases to extract aflatoxins deoxynivalenol, fumonisins, T-2, and ZEN, respectively, and incubates for 5 min. The recovery of 70–130% can be recorded. The iPOCT results can be captured via smartphones and uploaded to the RIDA®SMART APP database, and then are ready to be sent to e-mail, cloud, or printer via Wi-Fi and Bluetooth. Smartphone-based food safety high-throughput rapid test products, using six channels strip to achieve simultaneous qualitative detection of pesticide residues, including imidacloprid, acetamidine, carbendazim, difenoconazole, triazolone and thiamethoxam in tea soup. Six pesticides can be detected simultaneously each time, the test strip can be photographed through a smartphone, and the test data can be uploaded to the cloud for storage. The detection time was 8 min, significantly shorter than conventional chromatography-mass spectrometry. Currently, there are few iPOCT products, which require (1) the development of products suitable for complex substrates, (2) the expansion of detection targets, and (3) the quantitative stability of products.
Conclusions
The iPOCT in food safety has merits of on-site manner, portability, and universality. Thus, the iPOCT has triggered the coming generation for food safety, depending on the rapid progress in intelligent smartphone and applications. Here, we comprehensively discussed the optics and electricity based iPOCT in food safety. In addition, the rapid development of functional nanomaterials for iPOCT, like highly sensitive fluorescent labels or highly active nanomaterials for modifying electrodes, can provide a huge arena for iPOCT platforms with excellent performance in the real applications.
However, there are still difficulties in applying iPOCT. (1) Complex substrate: IPOCT is easy to detect only targets in water and food without color, but should be used for targets with the complex matrix. (2) Sample pre-treatment procedure: portability and simplicity are the most critical features of the iPOCT test, which also symbolizes that the sample pre-treatment process is very simple. Even one or two steps can be completed before entering the test. The development of the quick, easy, cheap, effective, rugged, and safe (QuEChERS) method has simplified the pre-treatment process for solid samples, which can effectively remove the original color of the food and other impurities that can affect the test results. However, using an acetonitrile solution of organic extract makes solid waste treatment one of the factors considered in the field detection of iPOCT. (3) iPOCT has high environmental requirements. For example, the storage of test strips in a 4℃ is preferred for a long time. During their storage, improper moisture and temperature can malfunction the antibody on cellulose acetate films. Thus, a stable and appropriate fabrication and storage conditions for test strips are recommended for reliable test results. Optical detection requires a cassette, which can avoid the influence of stray light. However, electrochemical devices are sensitive to temperature and need internal temperature compensation.
The future opportunities may be as follows: (1) Combining artificial intelligence with intelligent iPOCT data analysis is expected to complete the complex tasks of data statistics and analysis from multiple sources in order to explore greater value in food safety hazards. With artificial intelligence (AI) operating within a mature data science system, it can construct more advanced computer science and interdisciplinary systems. (2) High-throughput detection is expected to break through the simultaneous detection of harmful factors in complex matrices using iPOCT. Although current detection technology can simultaneously detect six pollutants, achieving high sensitivity, high throughput, and comprehensive screening for the same type of hazardous factor adapted to complex matrices has greater potential for implementation. (3) Standardization of detection technology is a key point in achieving interrecognition of the same type of iPOCT between different countries and regions. Standardizing iPOCT enables comparison of detection results across different people, different countries, and different fields. Food safety is related to the health and safety of the people. Through discussing the technology and commercialization of iPOCT, we aim to provide useful information for multidisciplinary studies such as food safety, analytical chemistry, nanomaterials, immunology.
Abbreviations
AChE: |
acetylcholinesterase |
AFB1: |
aflatoxin B1 |
AuNF: |
gold nanoflowers |
AuNP: |
gold nanoparticles |
BoNT: |
botulinum neurotoxin serotype |
CL: |
chemiluminescent |
CV: |
cyclic voltammetry |
DPA: |
biomarker dipicolinic acid |
DPV: |
differential pulse voltammetry |
H2O2: |
hydrogen peroxide |
Hg2+: |
mercury ions |
iPOCT: |
iIntelligent point- of- care test |
LM: |
latex microspheres |
LOD: |
limit of detection |
lqdb: |
labyrinth-like quantum dot nanosphere |
SWV: |
square wave voltammetry |
TAC: |
total antioxidant capacity |
ZEN: |
zearalenone |
Declarations
Author contributions
LZ: Writing—original draft. XY and ML: Writing—review & editing. ZT and ZZ: Conceptualization, Funding acquisition, Supervision, Writing—review & editing. All authors have read and agreed to the published version of the manuscript.
Conflicts of interest
The authors declare that they have no competing interests.
Ethical approval
Not applicable.
Consent to participate
Not applicable.
Consent to publication
Not applicable.
Availability of data and materials
Not applicable.
Funding
This research was funded by the
Copyright
© The Author(s) 2023.
References
- Malik A, Erginkaya Z, Erten H, editors. Health and safety aspects of food processing technologies. Springer Cham; 2019.
- Lee KM, Runyon M, Herrman TJ, Phillips R, Hsieh J. Review of salmonella detection and identification methods: aspects of rapid emergency response and food safety. Food Control. 2015;47:264–76. [DOI]
- Ren Y, Cao L, Zhang X, Jiao R, Ou D, Wang Y, et al. A novel fluorescence resonance energy transfer (FRET)-based paper sensor with smartphone for quantitative detection of Vibrio parahaemolyticus. Food Control. 2023;145:109412. [DOI]
- Liu S, He X, Zhang T, Zhao K, Xiao C, Tong Z, et al. Highly sensitive smartphone-based detection of Listeria monocytogenes using SYTO9. Chin Chem Lett. 2022;33:1933–5. [DOI]
- Riu J, Giussani B. Electrochemical biosensors for the detection of pathogenic bacteria in food. Trends Analyt Chem. 2020;126:115863. [DOI]
- Yu Y, Li Y, Zhang Q, Zha Y, Lu S, Yang Y, et al. Colorimetric immunoassay via smartphone based on Mn2+-mediated aggregation of AuNPs for convenient detection of fumonisin B1. Food Control. 2022;132:108481. [DOI]
- Zaczek-Moczydlowska MA, Beizaei A, Dillon M, Campbell K. Current state-of-the-art diagnostics for Norovirus detection: model approaches for point-of-care analysis. Trends Food Sci Technol. 2021;114:684–95. [DOI]
- Chansi, Chaudhary S, Mani A, Bharadwaj LM, Basu T. A mobile app integrated portable electrochemical sensor for rapid detection of organophosphate pesticides in vegetable extract. Materials Letters. 2022;309:131319. [DOI]
- Tang K, Chen Y, Tang S, Wu X, Zhao P, Fu J, et al. A smartphone-assisted down/up-conversion dual-mode ratiometric fluorescence sensor for visual detection of mercury ions and L-penicillamine. Sci Total Environ. 2023;856:159073. [DOI]
- Jiang X, Zhao Z, Liao Y, Tang C, Tremblay P, Zhang T. A recyclable colorimetric sensor made of waste cotton fabric for the detection of copper ions. Cellulose. 2022;29:5103–15. [DOI]
- Luo X, Huang G, Li Y, Guo J, Chen X, Tan Y, et al. Dual-modes of ratiometric fluorescent and smartphone-integrated colorimetric detection of glyphosate by carbon dots encapsulated porphyrin metal-organic frameworks. Appl Surf Sci. 2022;602:154368. [DOI]
- Rakkhun W, Jantra J, Cheubong C, Teepoo S. Colorimetric test strip cassette readout with a smartphone for on-site and rapid screening test of carbamate pesticides in vegetables. Microchem J. 2022;181:107837. [DOI]
- Qin T, Zhao X, Song C, Lv T, Chen S, Xun Z, et al. A ratiometric supramolecular fluorescent probe for on-site determination of cyfluthrin in real food samples. Chem Eng J. 2023;451:139022. [DOI]
- Cao Y, Liu Y, Li F, Guo S, Shui Y, Xue H, et al. Portable colorimetric detection of copper ion in drinking water via red beet pigment and smartphone. Microchem J. 2019;150:104176. [DOI]
- Umapathi R, Park B, Sonwal S, Rani GM, Cho Y, Huh YS. Advances in optical-sensing strategies for the on-site detection of pesticides in agricultural foods. Trends Food Sci Technol. 2022;119:69–89. [DOI]
- Rao H, Liu W, He K, Zhao S, Lu Z, Zhang S, et al. Smartphone-based fluorescence detection of Al3+ and H2O based on the use of dual-emission biomass carbon dots. ACS Sustainable Chem Eng. 2020;8:8857–67. [DOI]
- Xu C, Lan L, Yao Y, Ping J, Li Y, Ying Y. An unmodified gold nanorods-based DNA colorimetric biosensor with enzyme-free hybridization chain reaction amplification. Sens Actuators B Chem. 2018;273:642–8. [DOI]
- Chen H, Zhou K, Zhao G. Gold nanoparticles: from synthesis, properties to their potential application as colorimetric sensors in food safety screening. Trends Food Sci Technol. 2018;78:83–94. [DOI]
- Hwang J, Cho Y, Park MS, Kim BH. Microchannel fabrication on glass materials for microfluidic devices. Int J Precis Eng Manuf. 2019;20:479–95. [DOI]
- Lin C, Chao C, Lan C. Low azeotropic solvent for bonding of PMMA microfluidic devices. Sens Actuators B Chem. 2007;121:698–705. [DOI]
- Fahimi-Kashani N, Hormozi-Nezhad MR. A smart-phone based ratiometric nanoprobe for label-free detection of methyl parathion. Sens Actuators B Chem. 2020;322:128580. [DOI]
- Fan J, Yuan X, Li W, Zhou Y, Zhang J, Zhang Y, et al. Rapid and ultrasensitive method for determination of aflatoxin M1 in milk. Food Agric Immunol. 2020;31:849–58. [DOI]
- Lu Z, Chen M, Li M, Liu T, Sun M, Wu C, et al. Smartphone-integrated multi-color ratiometric fluorescence portable optical device based on deep learning for visual monitoring of Cu2+ and thiram. Chem Eng J. 2022;439:135686. [DOI]
- Hu S, Li D, Huang Z, Xing K, Chen Y, Peng J, et al. Ultra-sensitive method based on time-resolved fluorescence immunoassay for detection of sulfamethazine in raw milk. Food Agric Immunol. 2018;29:1137–49. [DOI]
- Hu X, Huang L, Wang S, Ahmed R, Li P, Demirci U, et al. Color-selective labyrinth-like quantum dot nanobeads enable point-of-care dual assay of Mycotoxins. Sens Actuators B Chem. 2023;376:132956. [DOI]
- Wu W, Zhou D, Chen X, Tang X, Jiang J, Yu L, et al. Intelligent point-of-care test via smartphone-enabled microarray for multiple targets: mycotoxins in food. Sens Actuators B Chem. 2022;360:131648. [DOI]
- Zeng Y, Li Q, Wang W, Wen Y, Ji K, Liu X, et al. The fabrication of a flexible and portable sensor based on home-made laser-induced porous graphene electrode for the rapid detection of sulfonamides. Microchem J. 2022;182:107898. [DOI]
- Ge Y, Li M, Zhong Y, Xu L, Lu X, Hu J, et al. Halloysite nanotube/black phosphorene nanohybrid modified screen-printed carbon electrode as an ultra-portable electrochemical sensing platform for smartphone-capable detection of maleic hydrazide with machine learning assistance. Food Chem. 2023;406:134967. [DOI] [PubMed]
- Park YM, Lim SY, Jeong SW, Song Y, Bae NH, Hong SB, et al. Flexible nanopillar-based electrochemical sensors for genetic detection of foodborne pathogens. Nano Converg. 2018;5:15. [DOI]
- Giordano GF, Vicentini MBR, Murer RC, Augusto F, Ferrão MF, Helfer GA, et al. Point-of-use electroanalytical platform based on homemade potentiostat and smartphone for multivariate data processing. Electrochimica Acta. 2016;219:170–7. [DOI]
- Xu Z, Liu Z, Xiao M, Jiang L, Yi C. A smartphone-based quantitative point-of-care testing (POCT) system for simultaneous detection of multiple heavy metal ions. Chem Engine J. 2020;394:124966. [DOI]
- Nixon EJ, Sakthivel R, ALOthman ZA, Ganesh PS, Chung RJ. Lanthanum nickelate spheres embedded acid functionalized carbon nanofiber composite: an efficient electrocatalyst for electrochemical detection of food additive vanillin. Food Chem. 2023;409:135324. [DOI]
- Yadav AK, Verma D, Lakshmi GBVS, Eremin S, Solanki PR. Fabrication of label-free and ultrasensitive electrochemical immunosensor based on molybdenum disulfide nanoparticles modified disposable ITO: an analytical platform for antibiotic detection in food samples. Food Chem. 2021;363:130245. [DOI]
- Nagabooshanam S, Roy S, Mathur A, Mukherjee I, Krishnamurthy S, Bharadwaj LM. Electrochemical micro analytical device interfaced with portable potentiostat for rapid detection of chlorpyrifos using acetylcholinesterase conjugated metal organic framework using Internet of things. Sci Rep. 2019;9:19862. [DOI] [PubMed] [PMC]
- Wu J, Tang X, Zhao S, Zhang Y, Ling C, Xing Y, et al. Microplasma and quenching-induced Co doped NiMoO4 nanorods with oxygen vacancies for electrochemical determination of glucose in food and serum. Food Chem. 2023;414:135755. [DOI]
- Shanmugam R, Koventhan C, Chen S, Hung W. A portable Ru-decorated cobalt phosphide on graphitic carbon nitride sensor: an effective electrochemical evaluation method for vitamin B2 in the environment and biological samples. Chem Eng J. 2022;446:136909. [DOI]