Abstract
Dry eye disease (DED) is a complex and multifactorial ocular surface disease affecting a large proportion of the population. There is emerging evidence of the impact of the microbiomes of the ocular surface and gut on the symptoms of DED, with many parallels being drawn to inflammatory diseases of other organ systems. A key factor involved in the promotion of healthy microbiomes, and which has been associated with ocular surface disease, is micro- and macronutrient deficiency. A comprehensive review of how these deficiencies can contribute to DED is absent from the literature. This review reports the composition of healthy ocular and gut microbiomes, and how nutrient deficiencies may impact these floral populations, with linkage to the subsequent impact on ocular health. The review highlights that vitamin B1 and iron are linked to reduced levels of butyrate, a fatty acid implicated in inflammatory conditions such as ulcerative colitis which itself is a condition known to be associated with ocular surface diseases. Vitamin B12 has been shown to have a role in maintaining gut microbial eubiosis and has been linked to the severity of dry eye symptoms. Similar beneficial effects of gut microbial eubiosis were noted with vitamin A and omega-3 polyunsaturated fatty acids. Selenium and calcium have complex interactions with the gut microbiome and have both been implicated in the development of thyroid orbitopathy. Further, diabetes mellitus is associated with ocular surface diseases and changes in the ocular microbiome. A better understanding of how changes in both the gut and eye microbiome impact DED could allow for an improved understanding of DED pathophysiology and the development of new, effective treatment strategies.
Keywords
Dry eye disease, microbiome, micronutrient, vitamin, dysbiosis, Sjögren’s syndrome, flora, uveitisIntroduction
Dry eye disease (DED) is a debilitating ocular surface disorder affecting up to 34% of the global adult population [1]. Symptoms include ocular discomfort, visual changes, and reduced quality of life; such that it is one of the most common reasons for ophthalmology referral [2, 3]. DED is a significant burden for patients. In the United States, the annual treatment cost of DED is 783 dollars per patient, this cost includes eyedrops, physician visits, in-clinic procedures, and nutritional supplementation [4]. Despite available therapies, many patients with DED continue to suffer and questions on pathophysiology remain unanswered [5].
Emerging evidence has linked dietary deficiencies to ocular surface diseases such as dry eye. For example, a large population study found that dietary deficiencies of macro- and micronutrients were associated with the presence of dry eye symptoms [6]. Guo et al. [6] found that patients with a high intake of vitamin B12, vitamin C, thiamine, polyunsaturated fats, and calcium reported a reduced likelihood of experiencing dry eye symptoms. This is supported by a recent small randomised controlled trial (RCT) comparing the intervention of a Mediterranean diet on measures of DED [7]. The diet, associated with high levels of polyunsaturated and omega-3 fatty acids, consisted of an increased intake of fruits, legumes, extra virgin olive oil, and nuts. Patients receiving the diet had significant improvements in their tear break up times (TBUTs), Schirmer’s tests, and Oxford staining scores [7]. Other evidence supports the link between diet and DED [8]. Kawakita et al. [9] randomised 27 dry eye patients to receive fish oil supplementation or placebo, with significant improvements in symptom experience, TBUT, and rose bengal staining score. More recently, Kawashima et al. [10] supplemented the diets of human patients with DED with a combined fish oil/micronutrient supplement, achieving similar results.
With a relationship between diet and microbiomes emerging it is of relevance to consider nutritional intake and its impact on DED [11]. To date, there have been no comprehensive reviews of this emerging relationship. In this review, the focus is on reporting the evidence supporting a relationship between diet and microbiomes, and secondarily, on considering the role of diet in DED. This review will inform clinicians in practice and may provide researchers with data able to inform the development of new therapeutics as well as an improved understanding of dry eye pathophysiology.
The ocular surface
The ocular surface encompasses a region including the cornea, conjunctiva, eyelid, and eyelashes, all of which are structures that contribute to the pathogenesis of DED [12]. DED is classified as aqueous-deficient, evaporative, or mixed in aetiology [13]. The most common type, evaporative dry eye, is associated with meibomian gland dysfunction (MGD), which leads to deficiencies in the tear lipid layer that under normal physiological conditions slows tear evaporation [14]. Aqueous deficient dry eye (ADDE) is characterised by failure of lacrimal tear secretion, which can be attributed to diseases that also affect conjunctival secretion such as primary and secondary Sjögren’s syndrome [15]. Sjögren’s syndrome is an autoimmune condition that results in lymphocytic infiltration and destruction of lacrimal glands which secrete the aqueous layer of the tear film consequently leading to ADDE [16]. The various forms of DED are associated with changes in the ocular surface microbiome.
The ocular surface microbiome
The ocular surface in healthy individuals has a microbiome composed of bacteria, viruses, and fungi [17]. The dominant ocular surface bacteria are Actinobacteria, Proteobacteria, and Firmicutes, accounting for over 90% abundance [18]. The most abundant viruses include multiple sclerosis-associated retrovirus (MSRV), human endogenous retrovirus-K (HERV-K), and the torque teno virus (TTV) [19].
The ocular surface microbiome will typically host commensal bacteria that do not cause inflammation or infection, rather contributing to homeostasis due to immunotolerance [20]. The presence of these normal ocular surface microbiota will inhibit the growth of pathogenic bacteria through competitive mechanisms. Modification of these ocular surface populations, such as higher abundance of Corynebacterium and Staphylococcus has been associated with MGD [21]. In the presence of non-immune tolerant organisms, the ocular surface epithelium membrane receptors present antigens to dendritic cells and lymphocytes consequently activating a defensive immune response [20].
A link between aberrant microbiota diversity and the presence of DED has been established [22]. In a clinical study, conjunctival swabs of patients with dry eyes demonstrated decreased microbiota diversity. Pseudomonas species were more abundant in healthy controls and Staphylococcus and Brevibacterium species more abundant in ADDE. An inverse relationship between microbiota diversity and the presence of ADDE has been also demonstrated in mice models [23]. ADDE has also been associated with mucin deficiency secondary to impaired goblet cell density [24]. A study culturing the ocular surfaces of 27 patients with varying grades of goblet cell dysfunction found a positive correlation between MGD grade and mean bacterial count, although the specific types of bacteria were not identified [25].
The ocular microbiomes of patients with MGD have been found to have greater microbial density and host more pathogenic microbes such as Corynebacterium macginleyi in comparison to surfaces with normal gland function [26, 27]. Furthermore, MGD is associated with chronic blepharitis which itself is a disease characterised by microbial proliferation and inflammation [28]. Propionibacterium sp., Corynebacterium sp., Streptophyta sp., Enhydrobacter sp., and Staphylococcus aureus strains from patients with chronic blepharitis produce lipolytic exoenzymes that can hydrolyse cholesterol esters and degrade lipids of the tear film [29–31]. The relevant studies investigating via DNA or RNA analysis the differences in the ocular surface microbiome between healthy controls and patients with DED are summarized in Table 1.
Studies reporting changes in the human ocular surface microbiome of DED state subtypes compared to healthy controls
First author | Year | Study type | DED type | Patients (N) | Significant alpha diversity change | Increased bacterial abundance or detection | Decreased bacterial abundance or detection |
---|---|---|---|---|---|---|---|
Andersson [22] | 2021 | Case-control | ADDE | 18 | Reduced | NR | 52 genera less abundant |
Zhang [32] | 2021 | Case-control | NR | 34 | Not significant | Pseudomonas Methylobacterium Bacteroides | Actinobacteria |
Zilliox [33] | 2020 | Case-control | NR | 8 | Reduced | Corynebacterium Staphylococcus | NR |
Li [34] | 2019 | Case-control | Combined | 35 | Reduced | Bacteroidia Bacteroidetes | Pseudomonas Pseudomonas plecoglossicida Pseudomonadaceae Gammaproteobacteria Proteobacteria |
Dong [21] | 2019 | Case-control | Evaporative | 47 | Not significant | Bacillales Proteobacteria Bacilli Staphylococcus Staphylococcaceae Firmicutes Staphlococcus epidermidis Alphaproteobacteria Gammaproteobacteria Sphingomonas Sphingomonadaceae Sphingomonadales Sphingomonas melonis | Actinomycetales Actinobacteria Corynebacteriaceae Corynebacterium |
Jiang [26] | 2018 | Case-control | EDE | 41 | Increased | Bacillus Micrococcus luteus Staphylococcus epidermidis Staphylococcus Microbacteriaceae | NR |
Graham [25] | 2007 | Case-control | NR | 12 | NR | Bacillus Propionbacterium acnes Klebsiella oxytoca | Erwinia |
Studies restricted to those employing RNA or DNA sequencing in lieu of culture only. EDE: evaporative dry eye; NR: not recorded
Changes to the ocular microbiome can also be induced iatrogenically in the management of DED and other ocular conditions. Due to its ocular surface toxicity, the common preservative benzalkonium chloride (BAK) used in certain lubricants has been linked to the symptoms of DED including irritation, redness, photophobia, and foreign body sensation [35]. It has previously been suggested that long-term exposure to BAK may be involved in the causation of DED [36] and BAK can induce DED in animal models [37]. In a recent study [38], the ocular microbiomes of unilateral glaucomatous eyes receiving glaucoma medication drops containing BAK were compared against contralateral eyes and the eyes of healthy controls. Several differences were found in BAK eyes, including increased alpha diversity, species abundance derangements with a propensity towards gram-negative organisms, poorer tear film measures, and a suggestion of increased synthesis of the pro-inflammatory endotoxin lipopolysaccharide. It is not yet clear how BAK, or glaucoma medications, contribute to this dysbiosis.
Gut microbiome and ocular disease
The dysbiosis of the gut eye axis has been hypothesised as a possible association for various ocular conditions such as diabetic retinopathy, age-related macular degeneration (AMD), choroidal neovascularisation, uveitis as well as Sjögren’s syndrome [39]. The composition changes of gut microbiota that have been associated with ophthalmic disease with DED manifestations are outlined in Table 2. Systemic diseases such as inflammatory bowel disease and rheumatoid arthritis (RA), have been associated with gut dysbiosis [40]. These disease states are well known to cause ocular inflammation in the form of uveitis, scleritis, episcleritis as well as conjunctivitis which may be associated with DED [40–42].
Studies reporting changes in the relative abundance of human gut microbiota associated with ophthalmic disease compared to healthy controls
First author | Year | Study type | Ophthalmic disease | Increased bacterial abundance | Decreased bacterial abundance |
---|---|---|---|---|---|
Moon [43] | 2020 | Case-control | Sjögren’s syndrome | Bacteroidetes | Actinobacteria Bifidobacterium Blautia Dorea Agathobacter |
Moon [43] | 2020 | Case-control | DED | Veillonella | Subdoligranulum |
Huang [44] | 2018 | Case-control | Acute anterior uveitis | Veillonella | Roseburia Lachnospiracea incertae sedis Dorea Blautia Clostridium XI Clostridium sensu stricto Odoribacter |
Huang [45] | 2021 | Case-control | Diabetic retinopathy | Blautia Bifidobacterium Lactobacillus | Escherichia-Shigella Faecalibacterium Eubacterium hallii group Clostridium |
Li [46] | 2022 | Case-control | Vogt-Koyanagi-Harada | Pseudomonas Stomatobaculum Lachnoanaerobaculum | Slackia Gordonibacter |
Wang [47] | 2023 | Cases compared against public dataset | Alistipes | Dorea | |
Wang [47] | 2023 | Cases compared against public datasets | Behcet’s disease | Bilophila Stenotrophomonas | Dorea Blautia Coprococcus Erysipelotrichacaeae Lachnospiracaeae |
Su [48] | 2020 | Case-control | Grave’s orbitopathy | Bacteroides Alistipes Prevotella | Firmicutes Proteobacteria Verrucomicrobia Tenericutes |
Gut microbiome analysis for Sjögren’s syndrome subjects compared to controls demonstrated reduced levels of bacteria including Bifidobacterium, Dorea, and Blautia, which are known producers of the anti-inflammatory short-chain fatty acid, butyrate [43, 49]. Conversely, Sjögren’s syndrome gut microbiome analysis also showed an increased abundance of members of the Prevotella, Odoribacter, and Alistipes compared to patients with non-Sjögren’s syndrome related DED. In a murine model investigating age-related gut dysbiosis and DED, older C57BL/6 mice had increasing levels of dry eye severity [50]. Gut microbiome analysis, after adjusting for age, revealed that higher corneal staining scores were significantly associated with an increasing abundance of Alistipes and Bacteroides and a decreasing abundance of Paraprevotella.
Furthermore, gut dysbiosis can dysregulate immune responses causing mucosal barrier dysfunction. This can lead to the translocation of pathogenic microbes and metabolites through the epithelial barrier, resulting in systemic inflammatory systemic conditions, and is hypothesised to be a factor in the pathogenesis of ophthalmic diseases such as AMD [51].
Micro- and macronutrients, the ocular surface microbiome, and ocular surface disease
There is emerging evidence that the ocular surface microbiome may be influenced by micro- and macronutrients such that diet may have an influence on ocular surface disease. Specific examples that support this hypothesis are discussed below (Figure 1).
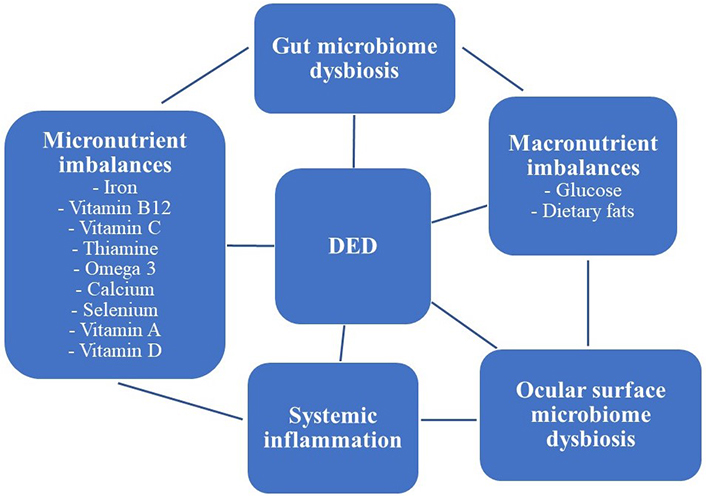
Visual graphic highlighting the complex relationship between diet, altered microbiomes, systemic inflammatory conditions, and DED
Iron
Iron is one of the most common nutritional deficiencies globally and is essential for several diverse cellular functions, particularly in the synthesis of haemoglobin and myoglobin for oxygen transportation [52]. Outside of this function, iron is also involved in systemic inflammation, with free iron being sequestered into storage as a defence mechanism to inhibit micro-organism growth [53]. This leads to a state of relative iron deficiency anaemia [54].
Regarding an effect on microbiota, iron deficiency results in significantly decreased butyrate concentrations and populations of the butyrate-producing bacteria Roseburia intestinalis in the gut [55]. Butyrate, a four-carbon short-chain fatty acid produced through the microbial fermentation of dietary fibres, plays a complex role in the colonic epithelium, promoting both an anti-inflammatory and anti-neoplastic colonic state [56–59]. A key mechanism of butyrate’s anti-inflammatory activity is its inhibitory effect on nuclear factor kappa B (NFkB) [60]. The NFkB pathway modulates the transcription of pro-inflammatory cytokines tumour necrosis factor-alpha (TNF-α) and interleukin-1 (IL-1) [61]. Butyrate is also a histone deacetylase (HDAC) inhibitor, reducing the expression of numerous inflammatory ILs when applied to endothelial cells [62].
Butyrate and its benefits of inflammatory suppression have effects that target the ocular surface, suggesting that this is a highly relevant compound of interest [63]. In a murine model of oral antibiotic-induced gut dysbiosis, topical lipopolysaccharide—a pathological component of gram-negative bacteria was applied to mouse cornea and conjunctiva. Expression of pro-inflammatory corneal cytokines was reduced in control models, with the authors hypothesising this response to be ameliorated by short-chain fatty acids such as butyrate [64]. Butyrate has also been administered topically to the cornea in mice models, with the effect of blocking the nucleotide-binding oligomerisation domain-containing protein (NOD)-like receptor family pyrin domain containing 3 (NLRP3) inflammasome pathway [65]. The inhibition of the NLRP3 pathway inhibits novel innate immunity signalling, hence decreasing inflammation and preserving corneal clarity following injury to the eye [66].
Vitamin B12
Vitamin B12 (cobalamin) is absorbed by both passive diffusion and receptor-mediated endocytosis in the intestine. It is required by methionine synthase and methylmalonyl-coenzyme A (CoA) mutase, which are necessary for biological methylation modifications of proteins or nucleic acid, and mitochondrial metabolism [67]. In a vitamin B12 deficient state often linked with reduced gut microbial diversity, there is a well-established association with inflammatory conditions such as ulcerative colitis and Crohn’s disease. The ophthalmic complications of inflammatory bowel disease are well recognised and can include secondary Sjögren’s syndrome along with the more common episcleritis, occurring in 0.3–5% of patients [68, 69].
Intestinal microbiota may either produce (20% of bacteria) or consume (80% of bacteria) vitamin B12 and this is dependent on the presence of specific bacterial species. It is essential for the homeostasis of these organisms to allow for a balance of vitamin B12 availability and production [70]. Vitamin B12 producers such as Lactobacillus reuteri and Enterococcus faecium have been implicated in improving vitamin B12 utilisation. Intestinal microbiota may also affect the bioavailability of vitamin B12 by influencing absorption-related physiological factors [71, 72]. Interestingly, over-supplementation of vitamin B12 in mice models results in a decrease in glucose utilising genes of Citrobacter rodentium, a decrease in Lachnospiraceae populations, and reduced microbial alpha diversity, leading to increased pathogen virulence [73].
Our analysis of the dietary influence of micronutrients on dry eye symptoms from the Blue Mountains Eye Study found that patients with a higher intake of vitamin B12 were 37% less likely to report one or more symptoms of DED [6]. They were additionally 36% less likely to report two or more symptoms of any severity. Other evidence for the benefit of vitamin B12 on the ocular surface includes studies showing a reduction in dry eye symptoms from nebulised vitamin B12, as well as improvements in DED with neuropathic corneal ulcer pain after the administration of parental vitamin B12 [74, 75]. The mechanisms behind vitamin B12 associated with improved DED are not certain, however there are several postulated processes. First, DED has been linked with a disequilibrium of reactive oxygen species (ROS) and the physiological antioxidative mechanisms, leading to a state of oxidative stress and inflammation [76]. There is evidence supporting vitamin B12 possessing antioxidant properties [77], with one study of combined vitamin B12 and hyaluronic acid drops reducing the level of oxidative stress in patients with dry eye. Second, in a rat model topical vitamin B12 has been suggested to improve corneal re-epithelialisation following injury, with the hypothesis that vitamin B12 assists in the synthesis of neurotrophic factors [78, 79].
Vitamin C
Vitamin C, also known as ascorbic acid, is an antioxidant and anti-inflammatory involved in the regulation of various transcription factors and signalling cascades required for immune cells to perform biological functions [80]. It cannot be endogenously synthesised, so dietary intake from the consumption of fruit and vegetables is needed to maintain adequate levels. As a reducing agent and specifically a sequential electron donor, vitamin C is a well-studied suppressor of oxidative stress [81].
Supplemental vitamin C has been studied in relation to gut microbiota. In a clinical trial, the beneficial effects of supplemental vitamin C included a greater abundance of bacteria Lachnospiraceae and a decrease in Bacteroidetes, Enterococcus, and Gemmiger formicilis [82]. In other studies, many of these findings were confirmed with a further emphasis on Bifidobacterium [83, 84]. Overall, these bacteria have a positive effect on gut barrier function and have anti-inflammatory properties [85]. Lachnospiraceae are important butyrate-producing bacteria that have also been shown to decrease in number in the setting of ocular inflammatory conditions such as Bechet’s disease and non-infective uveitis [49]. It is hypothesised that this decrease, as well as other gut microbial population changes, may be linked to a functional role in the disease progressions of Behcet’s and Vogt-Koyanagi-Harada which can be associated with DED [41, 47, 86]. In one case-control study, 100% of Vogt-Koyanagi-Harada patients (16/16) had abnormalities in the ocular surface suggestive of keratoconjunctivitis sicca [87]. Interestingly, in the faecal metagenomic study [87] distinct gut microbial signatures of both uveitides were found.
As well as being a potent antioxidant, vitamin C is beneficial to the cornea in several ways. Firstly, high concentrations of vitamin C in the corneal epithelium absorb ultraviolet radiation, helping protect the collagen of the cornea, and other posterior structures from radiation-induced damage [88, 89]. Vitamin C is also a necessary component of the healing process for damaged corneal stroma [90]. A regulator of collagen synthesis and the extracellular matrix, vitamin C promotes corneal wound healing with one study finding corneal opacification size after infectious keratitis was significantly improved following vitamin C supplementation [90]. Vitamin C’s benefits are not limited to the stroma, there is evidence that it improves the clonal ability of epithelial cells to expedite corneal epithelial wound healing [91]. Maintaining the corneal epithelium is relevant in treating DED, given its associations with symptomatic epithelial erosions and a thinner epithelium compared to healthy eyes [92, 93].
Thiamine (vitamin B1)
Thiamine is a water-soluble essential vitamin important to the metabolism of carbohydrates and branched-chain amino acids through glycolysis and the tricarboxylic acid cycle [94]. There is a hypothesised oxidative protection of ocular tissue with thiamine supplementation due to its role in immunometabolism [95, 96]. In thiamine-deficient settings, there is a decrease in populations of Roseburia rectale and Eubacterium rectale bacteria, while growth is optimised for Subdoligranulum variabile, Eubacterium rectale, Ruminococcus intestinalis, and Roseburia inulinivorans species [71]. Supplemental intake of thiamine has similarly been correlated with an increase in gut Ruminococcaceae, a microorganism which lacks the ability to synthesise its own thiamine [97]. Bacteria belonging to this family are involved with butyrate production, with levels of butyrate decreased in vitamin B1 deficient mice models. Gut-derived butyrate has a suppressive effect on ocular surface inflammation, including the disease process of DED [63]. Overall, the effect of gut dysbiosis in systemic inflammatory disease has been established such that there may be a role for dietary thiamine in the pathogenesis of dry eye via alterations in the gut microbiome.
Omega-3 polyunsaturated fatty acids
Dietary and supplemental effects of omega-3 polyunsaturated fatty acids (PUFAs) on gut microbiota have been described in several studies [98, 99]. While omega-3 PUFAs may be synthesised by the body via the elongation and desaturation pathways of α-linolenic acid, the most efficient manner of intake is dietary [100]. Omega-3 PUFAs are often associated with anti-inflammatory and autoimmune effects in conditions such as RA, ulcerative colitis, and lupus erythematosus [101, 102]. A meta-analysis of multiple RCTs concluded that omega-3 PUFA supplementation in RA reduced non-steroidal anti-inflammatory drug (NSAID) use, with a trend towards reduction in other markers including morning stiffness and swollen joint count. There are numerous complicated mechanisms by which omega-3 PUFAs may reduce inflammation. One notable mechanism is a change in gene expression secondary to alterations in transcription factor activation [103], which may occur as omega-3 PUFAs are natural ligands.
Dietary PUFAs positively modulate the host gut microbiota ecosystem by increasing the Firmicutes/Bacteroidetes ratio and decreasing the population of Faecalibacterium, which may have anti-inflammatory pathways and ameliorate intestinal permeability [104]. A decrease in inflammation has been correlated with the promotion of health-promoting bacteria such as Lactobacillus and Bifidobacterium in various health conditions secondary to PUFA supplementation [105].
In the Blue Mountains Eye Study, higher omega-3 fatty acid intake was associated with a reduced likelihood of reporting two or more dry eye symptoms [6]. This is consistent with existing literature [106], as evidenced by the findings of a recent meta-analysis and systematic review on the usage of omega‐3 fatty acids in the management of DED. Four outcomes of DED were measured, namely the Ocular Surface Disease Index (OSDI) score, TBUT, corneal staining, and Schirmer’s score. It is important to note that there was considerable heterogeneity between the studies and only an improvement in the OSDI was found after omega-3 PUFA supplementation. Theories of the mechanism of omega-3 PUFAs amelioration of dry eye symptoms focus on their neuromodulatory effects on corneal nerves as well as their ability to alter the fatty acid composition and therefore the properties of meibomian gland secretions [107]. This change may be beneficial in tear stabilization and may prevent inflammation from blockages in the meibomian gland ducts and meibum stagnation [108].
Calcium
Calcium is a mineral absorbed predominantly in the small intestine, enhanced by the effect of vitamin D, and essential for bone density [109]. It may be ingested through food or dietary supplements, with homeostatic regulation of circulating calcium mediated by an endocrine feedback system from the parathyroid gland [110]. The effect of a calcium-rich diet is proposed to have beneficial effects on inflammatory intestinal disorders, with a high level of interest in drugs which target the calcium-sensing receptor to decrease disease burden [111].
The effect of calcium supplementation on the gut microbiome is an area of relatively novel research [112]. In one rat model [113], calcium supplementation produced different effects on the gut microbiome depending on whether the calcium was paired with the prebiotic inulin or lactose. Inulin paired calcium increased intestinal concentrations of short-chain fatty acids, and the abundance of microbes such as Acinetobacter and Propionibacterium [113]. A different rat model found largely no difference between the effects of the prebiotics and echoed the previous study’s findings that calcium supplementation increased intestinal short-chain fatty acids such as propionic acid [114]. In that study, lactobacilli populations were notably increased. Growth of these bacteria in gut populations has shown beneficial effects on the symptoms of inflammatory bowel disease and overall immune function in the elderly [115, 116].
Recent research has linked gut levels of propionic acid to Graves’ disease [48]. Via analysis of the gut microbiomes of patients with Graves, it was discovered that reduced levels of propionic acid secondary to gut dysbiosis lead to a decrease in the number of regulatory T cells and vice versa for pro-inflammatory T-helper 17 (Th17) cells. It is suspected that this mechanism may contribute to the aetiology of Graves’ disease, which has implications for ocular health given the close association with Graves’ orbitopathy and DED [117, 118].
Selenium
Selenium is an essential mineral with antioxidant, anti-inflammatory, and immune function [119]. It is available in both food and supplemental form, with critical roles including antioxidation and anti-inflammation [119]. A deficiency of selenium has an established negative impact on immune cell activation, differentiation, and proliferation due to lower T-cell receptor (TCR) activation of CD4+ cells and consequently phagocytosis of antigenic organisms [120].
Selenium has a complex relationship with gut microbiota. Selenium is taken up by both the intestinal epithelium and gut microflora. There is a suggestion from murine studies that microflora will preferentially sequester selenium when supply is limited, reducing its availability to the host [121]. Microflora may also act as a buffer to reduce the toxicity of high selenium levels [122]. Deficiency of selenium increases the proliferation of bacteria such as Dorea sp. which are tied to the unique microbiome of patients with the inflammatory condition multiple sclerosis [123, 124].
Low selenium has been linked to ophthalmic disease. Selenium was discovered to be an integral part of many thyroid proteins, including iodothyronine deiodinases which activate thyroid hormone [125]. Due to this key function, low selenium has been associated with thyroid diseases such as Graves’ and subsequently thyroid eye disease, with evidence supporting selenium supplementation in patients with mild orbitopathy [126]. This is particularly relevant for the ocular surface with at least 65% of patients with thyroid eye disease experiencing concurrent DED [127]. Moreover, the ocular microbiomes of these patients are significantly altered compared to controls [128]. The as well diminished abundance of commensal surface flora such as Corynebacterium (10.1% vs. 16.9%), the ocular surface microflora of thyroid eye disease patients featured relative growth of pathogenic bacteria. This includes the Bacillus genera (1.7% vs. 0.3%) which is significant clinically due to the almost 50% rate of globe loss in Bacillus endophthalmitis [129].
Vitamin A
Vitamin A is a fat-soluble micronutrient responsible for cellular differentiation, epithelial barrier function, and immune function [125]. It has a well-known role in epithelial keratinisation, stratification, differentiation, and maturation, which is important as the cornea is lined with epithelium and is an important barrier to pathogen defence [130]. There are three types of vitamin A, with the retinoic acid form playing the most crucial role in the immune system [125]. The presence of retinoic acid induces activation and homeostasis for macrophages, T cells, and B cells. Retinoic acid has also been used as a therapeutic in DED and implicated in MGD pathogenesis [131].
In the setting of severe vitamin A deficiency, xerophthalmia, an ocular condition involving corneal xerosis which may progress to severe visual loss, highlights the importance of this vitamin [132, 133]. There is also a direct effect on microbiota, with a deficiency leading to decreased diversity of microbiota and increased populations of pathogenic bacteria such as Enterococcus faecalis [85]. In the setting of supplementation, there is evidence that the proportion of Bacteroidetes/Bacteroidales significantly increases with Bifidobacterium populations also decreasing [134]. Both these mechanisms would lead to a pro-inflammatory systemic effect.
Vitamin D
Vitamin D is a fat-soluble steroid hormone well recognised for the role of its metabolites in calcium homeostasis [135]. Many other functions and associations of vitamin D have been identified in recent years including relationships with gut microbiota, modulating innate and adaptive immune function, and inflammation [136–138]. For example, the vitamin D metabolite calcitriol suppresses cyclooxygenase-2 (COX-2) and subsequent prostaglandin (PG) production which acts to reduce the inflammatory response [139]. Additionally, low vitamin D has been linked to the loss of the ability of colonic epithelial to protect against bacterial ingress [140]. Mice fed a low vitamin D diet had reduced expression of the anti-microbial protein angiogenin-4 on histochemistry, after induction of a colitis episode [141]. These mice were also found to have highly proliferative intestinal microbiota, with up to 50 times the concentration of bacterial load compared to controls. It was hypothesised that vitamin D deficiency could increase the susceptibility of mice to inflammatory bowel disease secondary to impaired innate antimicrobial function. Compounding the issue for patients with inflammatory bowel disease is the fact that vitamin D has a complex absorption mechanism [142], and inflammatory bowel disease patients may have impaired absorption secondary to bowel resections. A 1991 study found a direct correlation between the length of bowel resected in Crohn’s patients and their ability to absorb vitamin D [143].
Vitamin D deficiency is also associated with ocular surface pathology such as Sjögren’s syndrome [144]. A meta-analysis of 10 observational studies of vitamin D levels compared against DED concluded that patients with dry eye have significantly lower serum vitamin D [145]. It was postulated that vitamin D deficiency could promote ADDE instead of evaporative dry eye, as Schirmer’s test between the groups was significantly different whilst the TBUT scores were comparable. Further, patients with dry eye syndrome refractive to standard administered high doses of intramuscular cholecalciferol had improvements in all outcome measures, albeit only short-term [146].
Glucose
Diabetes mellitus is a complex disease characterised by chronically impaired glucose control. It has been linked to many microbiome dysbiosis including in the eye [147], oral cavity [148], skin and feet [149, 150], lung [151, 152], and gut [153]. Diabetes mellitus has an established role in the pathogenesis of numerous ocular surface diseases [154] such as DED with symptom incidence positively correlated to the percentage of glycosylated haemoglobin [155, 156].
Studies have highlighted the differences in the ocular surface flora of diabetic patients [147, 157–163]. Diabetic conjunctival cultures are significantly more positive and have a higher prevalence of pathological organisms such as Staphylococcus aureus. This is clinically relevant because of the risk of introducing these organisms into the eye via intraocular procedures and may be one of the reasons why diabetic patients, in addition to their impaired immune function, have higher rates of post operative endophthalmitis [164].
Drawing parallels to dry eye symptoms, the severity of ocular microbiome dysbiosis is also suggested to be related to the severity of diabetic ophthalmic disease [147]. A recent study used next-generation sequencing analysis to examine the floral differences between diabetic and non-diabetic eyes with the addition of subgroup analysis between patients with varying degrees of diabetic retinopathy, as well as stratification of haemoglobin A1c (HbA1c) levels. Beta-diversity analysis revealed diabetic retinopathy, both proliferative and non-proliferative, as well as an HbA1c greater than 7 was associated with greater microbial diversity compared to control patients. Diabetic eyes were associated with more pathogenic organisms, for example, the gram-negative family Enterobacteriaceae, associated with keratitis [165] and endophthalmitis [166].
There is evidence that patients with diabetes mellitus have lower serum vitamin C levels, often at an average of 30% lower [167–169]. It is not clear whether a link can be drawn between these lower levels and the deleterious associations of vitamin C deficiency on the ocular surface discussed above.
The gut-eye axis and the treatment of DED
Contemporary management of dry eye addresses multifactorial causes of the condition. These include: artificial tears, topical ointments, punctual plugs, topical cyclosporin, topical corticosteroids, topical tacrolimus, and oral omega-3 supplementation [170, 171]. As previously discussed, the Blue Mountains Eye Study for micro- and macronutrients demonstrated specific dietary associations with dry eye. This included a reduced propensity to report two or more dry eye symptoms for patients who had higher oral intakes of vitamin C, vitamin B12, vitamin B1, polyunsaturated fats, and calcium. This suggests a connection between the gut microbiome and a possible effect on the ocular microbiome or the gut-eye axis.
Given the high number of micro- and macronutrients implicated in DED, there are varying tiers of evidence to support their supplementation as a treatment modality. Omega-3 PUFAs are the most studied supplement. Eight RCTs have been performed investigating their role in the treatment of treat DED and were recently analysed by O’Byrne and O’Keefe’s meta-analysis [106]. Despite substantial heterogeneity, the conclusion of the analysis was that omega-3 supplementation improves the subjective symptoms of DED. There are comparatively fewer clinical trials to support supplementation of the other listed micronutrients in this review. There exists one RCT on vitamin D supplementation in DED [172]. One hundred patients with concurrent dry eye and vitamin D deficiency were randomised to receive vitamin D supplementation in combination with artificial tears, or standard therapy alone for eight weeks. The three objective outcome parameters of DED the study examined all showed significantly greater improvement in the vitamin D supplemented group. This supports the large number of observational studies which have suggested a link between vitamin D deficiency and DED [173]. Oral vitamin B12 and thiamine were administered as a combined supplement in an RCT by Ren et al. [174] in a total patient cohort of 152 eyes, finding significant improvements in both subjective and objective outcome measures. However, the authors acknowledged it was not investigated whether the patients were deficient in vitamin B12 or thiamine before treatment, and the impact that could have.
There are fewer studies, and no RCTs, to support the oral supplementation of vitamin A [175]. An antioxidant supplement containing vitamins A and C (amongst others including herbal extracts) was trialled in a group of 20 patients with DED and compared against a placebo control [176]. Subjective and objective improvements in dry eye outcomes were reported; however, the external validity is limited as there was no separation of the individual antioxidants.
A different micronutrient not covered in the Blue Mountains Eye Study which could have potential benefit in DED is alpha-lipoic acid, a mitochondrial metabolic cofactor [177]. This is an area of research yet to be explored. Furthermore, it is of note that there is evidence suggesting DED can be aggravated by significant dietary alteration. Wu et al. [178] found that a high-fat diet in mice was associated with decreased tear production, increased epithelial staining, and high levels of factors regulating surface oxidative stress. This may indicate a further dietary risk factor for the development of DED.
Although there are many approaches to alleviate the symptoms of dry eye, there is no cure, and ongoing management is typically required [179]. In a survey of patients treated for dry eye, only 44% reported improvement, 19% had visual symptoms, and 24% experienced worsening ocular surface symptoms [180]. Utilising an understanding of the gut-eye axis with dietary modification as an additional tool to treat dry eye is potentially important [181]. Further investigation should be undertaken to clarify the relationship between the gut and the ocular surface microbiomes, and whether an alteration in the commensal flora is directly correlated to a change in ocular surface flora and vice versa.
Interestingly, alteration of the gut microbiome may be relevant in immune mediated dry eye. In the literature, there is only one study investigating the effect of faecal microbial transplantation (FMT) for the treatment of ophthalmic disease, namely Sjögren’s syndrome. This preliminary study performed faecal transfer for 10 patients with Sjögren’s syndrome or positive early Sjögren’s markers. The microbiota of these patients featured an abundance of Alistipes and Blautia, which is curiously dissimilar to a previous investigation of Sjögren’s syndrome gut microbiota [43]. FMT resulted in altered gut microbiomes for eight of the ten patients in early follow-up. Whilst the average microbial profile eventually regressed towards the original biota, five subjects reported subjective improvements in the symptoms of their DED after a three-month follow-up [182]. In addition, certain bacterial profiles remained more similar to the donor material. Given the paucity of other FMT data for ophthalmic indications, it is worth mentioning that there is a case report of a patient with steroid dependant ulcerative colitis and recurrent allergic rhinitis who was treated with FMT [183]. The patient successfully ceased her steroid use, and of note, her symptoms of allergic rhinitis dissipated. Ocular symptoms were not mentioned, but given the close atopic links between allergic rhinitis and conjunctivitis, this could also possibly be an area of future research [184].
Conclusions
This review highlights that there are clear correlations between DED, the microbiomes of both the gut and ocular surface, and diet. The ocular surface microbiomes of patients with DED often displayed increased alpha diversity, with a predisposition to opportunistic and pathogenic bacteria. Furthermore, alterations in the gut microbiome, which is influenced by dietary intake, were associated with a wide spectrum of ophthalmic diseases not purely limited to the ocular surface. Iron deficiency may lead to butyrate deficiency which decreases systemic inflammatory suppression, amongst other effects. Derangements in the intake of vitamin A, B1, B12, C, omega-3 fatty acids, calcium, and selenium are all associated with changes in the gut microbiome and linked to numerous ocular surface diseases. Associations of the translation between alterations of gut microbiota and those existing within the ocular surface need to be investigated, so that dietary or supplementation intervention may be a more objectively precise tool to utilize in managing dry eye. Beyond this, further investigation is warranted where individual dietary and supplementary factors are tested against the ocular microbiome and DED. Lastly, FMT for ophthalmic indications is an under-researched field, the exploration of which could lead to exciting discoveries in both the aetiology and treatment of ocular surface disease.
Abbreviations
ADDE: |
aqueous deficient dry eye |
BAK: |
benzalkonium chloride |
DED: |
dry eye disease |
FMT: |
faecal microbial transplantation |
MGD: |
meibomian gland dysfunction |
PUFAs: |
polyunsaturated fatty acids |
RA: |
rheumatoid arthritis |
RCT: |
randomised controlled trial |
TBUTs: |
tear break up times |
Declarations
Author contributions
MP and BG: Writing—original draft, Writing—review & editing. DL and SLW: Writing—review & editing. KGJO: Conceptualization, Writing—review & editing.
Conflicts of interest
The authors declare that they have no conflicts of interest.
Ethical approval
Not applicable.
Consent to participate
Not applicable.
Consent to publication
Not applicable.
Availability of data and materials
Not applicable.
Funding
Not applicable.
Copyright
© The Author(s) 2024.