Abstract
Lung cancer remains a leading cause of cancer-related deaths globally, and a significant number of patients are ineligible for surgery, while chemoradiotherapy often shows limited efficacy, a systemic distribution, a low drug concentration at tumor sites, severe side effects, and the emergence of drug resistance. In this context, a nanodrug delivery system (NDDS) has emerged as a promising approach for lung cancer treatment, offering distinct advantages such as targeted delivery, responsiveness to the tumor microenvironment, site-specific release, and enhanced induction of apoptosis in cancer cells, ultimately leading to tumor growth inhibition or even elimination. This review aims to provide an overview of the physiological characteristics of lung cancer, highlight the limitations of conventional treatment methods, and extensively examine recent significant advancements in NDDS utilized for lung cancer therapy. The findings from this review lay the foundation for further development and optimization of NDDSs in the treatment of lung cancer.
Keywords
Lung cancer, drug delivery system, nanotechnology, targeted therapy, cancer treatment, active targeting ligand, pharmacology, oncologyIntroduction
Lung cancer is the leading cause of cancer deaths worldwide, with approximately 2.2 million (11.4%) new cases and 1.8 million (18.0%) deaths reported in 2020 [1]. It is a highly heterogeneous disease with complex clinical effects and a poor prognosis [2]. Due to the lack of preventive measures and early diagnosis methods and the variability of the tumor environment, lung cancer is prone to high metastasis and recurrence rates and multidrug resistance (MDR) [3]. Therefore, improving the therapeutic effect on lung cancer is an urgent problem.
Histological studies of cancer cells have categorized lung cancer into two types: small cell lung cancer (SCLC) and non-SCLC (NSCLC), with SCLC accounting for approximately 15% and NSCLC accounting for approximately 85% of cases [4]. Although current medical technology can effectively intervene in pulmonary neoplasms to a certain extent through surgical resection, chemotherapy, and radiotherapy, improving the survival rate of lung cancer patients remains challenging [5].
Surgery is not suitable for all patients, as lung cancer is often diagnosed at an advanced stage and has a poor prognosis and high recurrence rate. Radiotherapy, on the other hand, has strong systemic side effects and can cause skin damage in the radiated area, limiting its therapeutic effect [6]. Chemotherapy, another main treatment for intermediate-stage disease, is hindered by poor water solubility, lack of targeting, and the first-pass effects of most drugs, resulting in low drug concentrations at the tumor site [7, 8]. As a result, current treatments are not highly effective.
However, due to the significantly higher growth rate of tumor tissues compared to normal tissues, defects between tumor vascular endothelial cells, wider gaps in the vascular wall, poor structural integrity, insufficient lymphatic drainage within the tumor, and a lower blood flow rate, macromolecular-like substances and lipid particles can selectively permeate and be retained in the tumor, which is known as the enhanced permeability and retention (EPR) effect [9]. This phenomenon provides opportunities for developing novel therapeutic approaches that exploit these characteristics.
Nano-based agents have the ability to overcome biological and chemical barriers within the human body. They can improve the pharmacokinetics and biodistribution of drugs and prevent early inactivation or biodegradation [10]. Additionally, they can be functionalized with modified targeting ligands, antibodies, and peptides to anchor them to receptor-overexpressing structures and specifically bind to cancer cells [11]. This enables active targeting, tumor site accumulation, and responsiveness to the tumor microenvironment (TME) or external stimuli such as temperature, light, and ultrasound, thus influencing these agents’ affinity or binding state, triggering the release of drugs, and promoting accumulation of therapeutic molecules in tumor tissues [12]. Moreover, the use of nano-based agents can reduce accumulation in normal organs, minimize systemic toxicity, and enhance treatment efficacy [13]. The use of nano-based carriers for drug delivery offers several advantages, including a high surface area-to-volume ratio, adjustable thermal, magnetic, optical, and electrical properties, an ability to synthesize diverse shapes and sizes, a high drug loading capacity, and stimulus-response sensitivity for precise spatiotemporal controlled drug release [14]. However, despite promising results in preclinical trials, the clinical translation potential of nano-based agents has not been fully realized due to challenges associated with reproducibility, large-scale manufacturing, and potential toxicological and safety hazards. In this study, we aim to leverage the physiological characteristics of lung cancer to clarify drug targets and behavioral processes in the tumor environment and thus provide insights for the design of more intelligent and successful drug delivery systems.
Physiological characteristics of lung cancer
Lung cancer is an intricate and diverse disease marked by the deterioration of lung epithelial cells [15]. Developing a profound understanding of the physiological attributes of the disease is essential to drive research in innovative diagnostic methods, treatments, and prognostic assessments. Targeted regulation of abnormal molecular signals and their subsequent pathways offers a promising avenue for the development of therapeutic interventions. To devise effective strategies for lung cancer treatment, elucidating the molecular pathogenesis, epigenetics, and signaling pathways mediated by pertinent molecules is imperative (Figure 1).
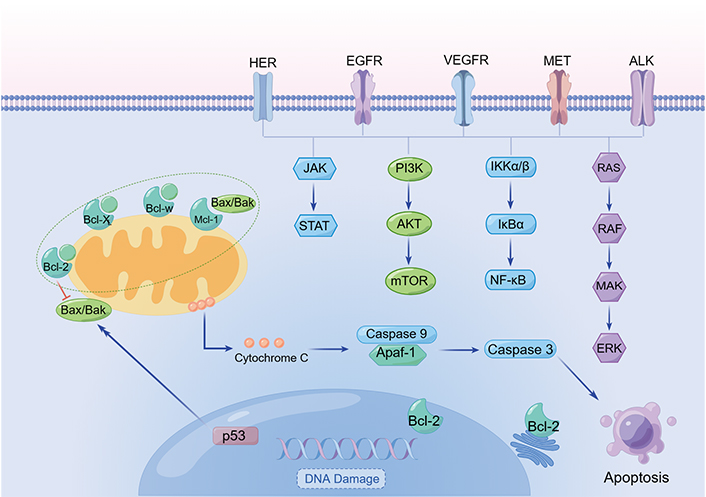
Typical signaling pathways in lung cancer. Created by Figdraw. The arrows mean the direction of signal transmission, except that before “apoptosis” means induction. AKT: protein kinase B; ALK: anaplastic lymphoma kinase; Apaf: apoptotic protease activating factor; Bcl-2: B cell lymphoma 2; Bak: Bcl-2 homologous antagonist/killer; Bax: Bcl-2-associated X protein; Bcl-XL: Bcl-extra large; EGFR: epidermal growth factor (EGF) receptor; ERK: extracellular signal-regulated kinase; HER: human EGFR receptor; JAK: Janus kinase; MAK: male germ cell-associated kinase; Mcl-1: myeloid cell leukemia 1; MET: mesenchymal-epithelial transition factor; PI3K: phosphatidylinositol 3-kinase; RAF: rapidly accelerated fibrosarcoma; RAS: rat sarcoma; STAT: signal transducer and activator of transcription; VEGFR: vascular endothelial growth factor (VEGF) receptor
Similar to other tumors, the development of lung cancer involves activation of growth-promoting proteins, such as EGFR, Kirsten RAS oncogene homolog (KRAS), and HER2. Notably, more than 50% of NSCLC patients who smoke and consume alcohol have p53 mutations, and certain compounds have shown potential for reactivating p53 for lung cancer treatment [16].
Lung cancer biomarkers, which are identified through chromosomal aberrations and mutations, often serve as attractive therapeutic targets. In this section, we will discuss the common variant types and clinical significance of proto-oncogenes, oncogenes, and molecular signaling pathways in lung cancer. Furthermore, we will explore mutated genes, specific ligands, and the tumor metastasis microenvironment associated with lung cancer, aiming to provide insights into the development of nanodrug delivery systems (NDDSs) for lung cancer [17]. By probing into the scientific understanding of lung cancer at various levels, researchers can pave the way for personalized and comprehensive treatment strategies that consider genetic, molecular, histopathological, and clinical factors, which holds promise for improving the diagnosis, treatment, and prognosis of lung cancer.
Occurrence of lung cancer
Lung cancer occurs mainly due to genetic mutations, including EGFR oncogenic mutations, ALK and ROS proto-oncogene 1-receptor tyrosine kinase (ROS1) fusion, and v-raf murine sarcoma viral oncogene homolog B1 (BRAF) mutations, which have been approved by the U.S. Food and Drug Administration (FDA) as therapeutic targets for NSCLC. In addition, KRAS mutations, aberrations in MET, which encodes the hepatocyte growth factor (HGF) receptor (HGFR), and mitogen-activated protein kinase kinase 1 (MEK1) overexpression in NSCLC may also be potential targets for the treatment of lung cancer [18].
EGFR is a member of the Erb-B2 receptor tyrosine kinase 2 (ErbB) family of transmembrane receptor tyrosine kinases (TKs). The ErbB receptor consists of an extracellular ligand binding domain, a transmembrane domain, and an intracellular TK domain [19]. Seven known ligands of EGFR induce different biological effects even in the same cell [20]. EGFR can induce signaling pathways, including the PI3K/AKT/mammalian target of rapamycin (mTOR) pathway, RAS/RAF/MEK/ERK pathway, and JAK/STAT pathway, which are involved in the pathogenesis of various tumors, including NSCLC [21]. Mutations in the EGFR gene affect tumor pathogenesis, including cell proliferation, survival, and differentiation, neovascularization, invasion, and metastasis [22]. Therefore, EGFR inhibitors are typically compounds that directly inhibit TK phosphorylation through physical interaction with ATP or enzyme-substrate binding sites. Third-generation TK inhibitors (TKIs), such as osimertinib, which has shown efficacy against EGFR T790M mutations, have been developed to overcome resistance to previous EGFR inhibitors.
HER2 is another receptor TK in the ErbB/HER family, which together with EGFR encodes a membrane-bound glycoprotein TK that binds to other ligands to form a heterodimer and activates downstream signaling [23]. HER2 amplifications and mutations are considered to be usually unrelated and clinically distinct driver alterations that can be used to segment lung adenocarcinoma patients for targeted therapy [24, 25], and the development of drugs targeting HER2 remains an urgent clinical need [26]. HER2 aberrations in lung cancer may be resistant to EGFR TKI therapy due to tissue specificity [27]. Currently, trastuzumab/paclitaxel (PTX) combination therapy has promising applications for the treatment of HER2-positive lung cancer.
The KRAS gene is a member of the RAS proto-oncogene family, which includes neuroblastoma RAS viral oncogene homolog (NRAS) and Harvey RAS viral oncogene homolog (HRAS). G proteins encoded by the KRAS gene play a key role in regulating cell proliferation, differentiation, and growth signal transduction pathways [28]. In normal resting cells, RAS protein binds to guanosine-5’-diphosphate (GDP) in an inactive state; when the upstream growth factor receptor is activated, RAS protein binds to guanosine triphosphate (GTP) and becomes active [29], which causes the protein to lose its intrinsic GTPase activity, preventing GTP from turning into GDP and thus resulting in the unchecked proliferation of the RAS/RAF/MEK/ERK signaling pathway downstream of EGFR [30]. Although KARS mutations are common in lung cancer, the results of clinical trials of relevant targeted drugs have been unsatisfactory, and further research is needed.
The BRAF gene encodes serine/threonine protein kinase, a downstream effector protein of KRAS [31]. BRAF proteins are RAS-RAF-MEK-activated ERKs that play a critical role in the MAPK/ERK signaling pathway and are involved in the regulation of cell proliferation and growth [32]. The therapeutic regimen of the BRAF inhibitor dabrafenib combined with the downstream MEK inhibitor trametinib for NSCLC with metastatic BRAF V600e mutation has been approved by the FDA and European Medicines Agency (EMA) [33]. Novartis has received approval from the State Drug Administration for a new indication for the treatment of BRAF V600 mutation-positive metastatic NSCLC with the dual-targeted combination of dabrafenib mesylate capsules and trametinib tablets.
ALK belongs to the insulin receptor superfamily [34], is activated by the family with sequence similarity 72 member A (FAM150A) and FAM150B ligands [35], and is expressed as a fusion gene in various cancers [36]. The ALK fusion protein dimerizes in a ligand-independent manner and activates the PI3K/AKT, RAS/RAF/MAPK1, and JAK/tyrosine aminotransferase (TAT) signaling pathways via the abnormal structure of the ALK TK. The abnormal constitutive activation of ALK TK leads to cell proliferation disorder and participates in the regulation of cell proliferation and apoptosis. To date, three ALK TKIs (crizotinib, ceritinib, and alectinib) have been used clinically for the treatment of ALK-abnormal NSCLC [37]. ALK TKIs often achieve significant tumor regression in patients with NSCLC with ALK rearrangements; however, in most cases, ALK TKI-resistant tumors’ likelihood of reemergence is high within a few years.
ROS1 encodes a receptor TK containing a large N-terminal extracellular region [38], a 3-hydrophobic one-way transmembrane region, and a C-terminal intracellular TK region [39, 40]. ROS1 regulates cell growth and apoptosis through the MAPK/ERK, PI3K/AKT, JAK/STAT3, and Src homology 2 domain-containing protein tyrosine phosphatase 1/2 (SHP1/2) signaling pathways [41]. All ROS1 fusions retain 14 intact ROS1 kinase structural domains [42]. Bilateral lung adenocarcinomas occur in transgenic mice expressing ezrin (EZR)-ROS1 in the alveolar epithelium [43]. Crizotinib, an ALK/ROS1/MET inhibitor, is an FDA-approved targeted agent for the treatment of advanced ROS1-rearranged NSCLC, and studies have shown that some ALK TKIs have dual inhibitory activity against ALK and ROS1 [44].
The MET gene encodes HGFR [45], which affects key processes such as cell proliferation, differentiation, motility, and tumor angiogenesis through the PI3K/mTOR, STAT, and MAPK signaling pathways [46, 47] and is closely associated with the development of various cancers [48]. However, MET exon 14 jump mutations and high levels of MET amplification have emerged as potential predictive biomarkers [49, 50]. Capmatinib (INC280) is a potent and selective MET receptor inhibitor that has shown antitumor activity with various MET activations and can cross the blood-brain barrier [51]. Monoclonal antibodies to the MET TKI onartuzumab in combination with the EGFR TKI erlotinib block HGF binding to MET receptors for second and third-line treatment of NSCLC after chemotherapy failure [52].
Lung cancer metastasis-related molecules
The main objective of systemic therapy for metastatic NSCLC is to reduce the burden of symptoms and improve the survival rate and quality of life [53]. Many cytotoxic chemotherapy regimens exhibit significant toxicities (e.g., alopecia, nausea), and the role of surgery and radiotherapy in prolonging disease-free survival is being studied for patients with lower metastatic tumor burdens. However, targets for drugs that are essential for tumor cell survival or immune evasion and can be tailored to patients’ tumor characteristics remain to be identified [54].
CD44 plays an important role in cell adhesion [55] and has a significant negative correlation with transfer potential [56]. The cell surface adhesion receptor CD44 is a positive regulator of programmed cell death ligand 1 (PD-L1) expression in NSCLC cells. CD44 activates PD-L1 transcription by cleavage of the intracytoplasmic domain. In one study, the proportion of PD-L1 tumors in patients with metastatic NSCLC was scored as 1% [57], providing a new rationale for CD44 as a key therapeutic target to inhibit intrinsic PD-L1 tumor function [58]. Effector T cell depletion is caused by decreased phosphorylation of various signaling molecules, such as ERK, Vav, and phospholipase Cγ (PLCγ) [59], which regulate T cell activation and proliferation through nuclear factors that activate T cells, leading to cancer immune evasion and promoting the growth, migration, and invasion of lung cancer cells [60].
The metastasis of lung cancer to the central nervous system (CNS) is the main clinical obstacle leading to the low 5-year survival rate of advanced diseases. Therapeutic drugs do not easily cross the blood-brain barrier, which substantially limits treatment and results in a poor prognosis. Therefore, the relevant driving factors and molecular mechanisms of tumor metastasis and targeted prevention and treatment require attention. Cytoskeletal proteins and motility-related genes such as metallothionein 2A (MT2A), fascin actin-bundling protein 1 (FSCN1), microtubule-associated protein 7 (MAP7), and chemokine-CXC-motif chemokine ligand 13 (CXCL13) were significantly upregulated in tumor metastases. CXC-motif chemokine receptor 4 (CXCR4) is expressed in 90% of primary tumors and 100% of brain metastases, and CX3C-motif chemokine receptor 1 (CX3CR1) is also a chemokine ligand associated with lung cancer and metastatic spread [61]. Overexpression of N-cadherin and decreased expression of kinesin family member C1 (KIFC1), E-cadherin, and BPTF/FALZ can predict brain metastasis [62]. HGFR expression is observed in approximately 30% of adenocarcinomas, and cellular-MET (c-MET) amplification is observed in approximately 10% of adenocarcinomas [63].
TME
The TME consists of extracellular matrix, soluble components (growth factors, chemokines, etc.), and cellular components (tumor cells, fibroblasts, endothelial cells, etc.). Tumor-associated macrophages (TAMs), vascular endothelial cells, and fibroblasts in the tumor matrix secrete growth factors and chemokines to attract and regulate the behavior of stromal cells and immune cells and promote tumor formation and growth. Tumor-derived cytokines such as IL-6, growth arrest specific protein 6 (GAS6), HGF and EGF can promote resistance to targeted therapy through autocrine signaling [64]. The interaction between tumor cells and the TME, such as changing cell adhesion by increasing the expression of cadherin and integrin β1 and reducing the expression of β2-microglobulin, affects the response of tumor cells to targeted therapy. Cytokines secreted by cancer-associated fibroblasts (CAFs) and mesenchymal stem cells (MSCs) in the matrix lead to EGFR TKI resistance by activating CXCR4 and IL-6R [65]. Due to an insufficient oxygen supply, tumor cells are able to metabolize energy only through anaerobic enzymes, resulting in lactic acid accumulation; meanwhile, ion exchange proteins on the tumor cell membrane are constantly transporting intracellular H+ outside the cell to avoid causing acidosis, resulting in a lower pH of the TME and an acidic environment, which will cause apoptosis and release cell debris and chemokines, leading to inflammatory cell infiltration and inflammatory factor secretion. However, the typical characteristics of the TME, such as hypoxia, low pH, and a high bioreduction environment, can be used as the conditions for stimulus-responsive drug release [66]. Chen et al. [67] found that hypoxia can improve the drug resistance of adriamycin in the treatment of NSCLC by inhibiting the expression of MDR-associated protein 1 (MRP1) and P-glycoprotein (P-gp) and enhancing the chemical sensitization of MRP1 and P-gp blockers.
Tumor angiogenesis and vascular abnormalities are critical to the growth and metastasis of solid tumors [68], and many proteins and small molecules have been demonstrated to be involved in angiogenesis, including VEGF, platelet-derived growth factor (PDGF), and fibroblast growth factor (FGF). Small-molecule TKIs, particularly multitargeted vascular kinase inhibitors, selectively inhibit downstream VEGFR pathway-mediated activation [69]. At present, various small-molecule antiangiogenic TKIs, such as apatinib, anlotinib, and nintedanib, have been used and evaluated in lung cancer clinical studies, as well as FGF receptor (FGFR) with TKIs alone or in combination with chemotherapy [70, 71].
NSCLC is immunogenic [72], and approximately two-thirds of lung tumor-infiltrating immune cells consist of T and B cells, with the remainder consisting of TAMs, neutrophils, a few dendritic cells (DCs) and natural killer (NK) cells [73], which are involved in antitumor responses [74]. A decrease in NK cells and enrichment of regulatory T (Treg) cells at tumor sites, where CD4+ Th1 cells, activated CD8+ T cells, and even γδ-T cells are frequently involved in type I immune responses, are associated with a good prognosis in lung cancer patients [75]. Immune checkpoint inhibitors (ICIs) have become the treatment of choice for recurrent or metastatic cancers, with the most widely used being anti-cytotoxic T lymphocyte associated protein 4 (CTLA4) and anti-programmed cell death protein-1 (PD1) antibodies [76]. CTLA4 is expressed on tumor-infiltrating T cells and is an inhibitory receptor whose main role is to regulate the degree of early T cell activation [77]. Anti-CTLA4 antibodies block inhibitory signals between antigen-presenting cells and T lymphocytes involving CTLA4 molecules. PD1 is highly expressed in Treg cells, and its main role is to inhibit T cell activation in peripheral tissues and suppress autoimmunity [78, 79]. Targeted immunoregulatory molecules, such as anti-4-1BB, Ox40, inducible T cell costimulator (ICOS), and CD40, block antibodies of lymphocyte-activation gene 3 (LAG3), B7-H4, Tim3, and killer Ig-like receptors (KIRs), and related therapeutic drugs are being developed. Since in vivo immune regulation involves complex regulatory pathways, combination immunotherapy will have a better therapeutic effect [80].
Ligands targeting tumor-specific receptors
In the context of cancer treatment, a successful cure often depends on achieving high concentrations of drugs specifically within tumor tissues. Systemic distribution of drugs throughout the body can lead to adverse effects and hinder the desired therapeutic outcomes. To overcome these limitations, nanocarriers have emerged as promising vehicles for drug delivery, offering improved pharmacokinetics and targeted delivery to tumors. Extensive research efforts have focused on designing nanoparticles with multiple functions to address the biological barriers encountered during intravenous administration. When nanoparticles are administered intravenously, they tend to be taken up by resident macrophages of the mononuclear phagocyte system (MPS) [81]. Consequently, significant accumulation of nanoparticles occurs in organs such as the spleen and liver, resulting in nonspecific distribution of nanotherapeutic drugs to healthy organs.
To overcome these challenges, identifying specific ligands that can selectively target receptors expressed by lung cancer cells is crucial. Active targeting strategies can be employed to achieve site-specific delivery of therapeutic drugs and facilitate their efficient accumulation in lung cancer tissues. By employing nanocarriers with active targeting capabilities, enhancing the specificity and efficiency of drug delivery to lung cancer cells becomes feasible. This approach holds significant potential for improving the therapeutic outcomes of lung cancer treatment while minimizing adverse effects on healthy organs.
Folate
The folate receptor (FR) family consists of four members, including FRα, FRβ, FRγ, and FRδ [82]. Silencing FR expression can inhibit the proliferation of cancer cells and promote apoptosis of human NSCLC cells [83]. FRβ has been demonstrated to be overexpressed in M2-polarized TAMs, and FR-positive TAMs are related to the poor prognosis of lung cancer [84]. By targeting FR overexpression of M2 TAMs and tumor cells, the proportion of M2 TAMs was reduced, and the proliferation and metastasis of tumor cells were inhibited, which had a synergistic effect on inhibiting tumors. Tie et al. [85] targeted FRβ-positive TAMs in lung cancer with folic acid-modified liposome complexes, which significantly promoted apoptosis of tumor cells and M2 TAMs. Clinical studies are currently assessing the efficacy of FRα combined with chemotherapy drugs and humanized anti-FRα antibodies [86].
Transferrin
The transferrin (Tf) family plays an essential role in transporting ferrum in blood after the formation of ferritin complexes and proliferation, differentiation, and even antioxidation. The expression of Tf receptor (TfR) in tumor cells is approximately 100 times that in normal cells. Tf is a glycoprotein that controls extracellular ferrum levels, reversibly binds multivalent ions, including copper, cobalt, and ruthenium [87], and is widely used as a targeting ligand. Coupling anticancer drugs with Tf can significantly improve selectivity and toxicity and overcome drug resistance, resulting in better therapeutic outcomes [88]. Lu et al. [89] designed self-assembled DNA and Tf to form a dual-targeted ruthenium complex with antitumor and antimetastatic properties that inhibits tumor growth and prevents lung metastasis by acting on Tf/TfR.
iRGD
VEGF, αvβ3 integrin, matrix metalloproteinase (MMP), and vascular cell binding molecule-1 (VCAM-1) are overexpressed in tumor vascular endothelial cells. Using the tumor homologous peptide iRGD (CRGDKGPDC) as the binding agent, a peptide-mediated delivery strategy for compounds penetrating into the tumor parenchyma was developed [90]. Combined with iRGD, the sensitivity of tumor imaging agents can be significantly improved, which is helpful for deep tumor penetration in the preparation and delivery of therapeutic molecules to the target site and enhancement of the activity of antitumor drugs. Su et al. [91] designed a dual-targeted combination drug delivery system based on multiwalled carbon nanotubes (MWNTs) for the antiangiogenic treatment of lung cancer, which showed significant tumor growth inhibition in A549 cells and xenograft nude mice.
β2-adrenergic receptor agonists
β2-adrenergic receptors (β2-ARs) are highly expressed in bronchial smooth muscle and the lung. Different β2-AR agonists, such as formoterol and salmeterol, have been used as targeted ligands to enhance receptor-mediated delivery to the lungs [92]. β-AR belongs to the G-protein-coupled receptor (GPCR) family and is subdivided into three different subtypes: β1, β2, and β3 [93]. The binding of β2-AR agonists to GPCRs leads to the internalization of receptor/ligand complexes through a process mediated by globulin [94]. Elfinger et al. [95] used the β2-AR agonist clenbuterol (Clen) to improve the gene transfer efficiency of the polyethylenimine (PEI) gene vector in vitro in alveolar epithelial cells and in vivo in the mouse lung, which led to the uptake of clenbuterol-specific cells mainly into alveolar epithelial cells, indicating that the coupling of β2-AR ligands to nonviral gene vectors is a promising method to improve gene delivery to the lungs.
Tumor necrosis factor-related apoptosis-inducing ligand
Tumor necrosis factor-related apoptosis-inducing ligand (TRAIL) is an apoptotic target currently under exploration for cancer drug development. TRAIL, a member of the tumor necrosis factor (TNF) receptor superfamily, is a type II transmembrane protein that can induce cancer cell apoptosis [96]. As a stable soluble trimer, this protein selectively induces apoptosis in many cancerous cells but does not induce apoptosis in normal cells. Therefore, TRAIL can be used to selectively target cancer cells and has attracted widespread attention as a potential tumor-specific cancer therapeutic agent [97]. Interferon (IFN) activates Apo2 ligand (Apo2L)/TRAIL transcription through specific regulatory elements in its promoter and may be involved in the activation of NK cells, cytotoxic T lymphocytes, and DCs [98]. The activated antibody against the TRAIL receptor and a soluble truncated TRAIL ligand are in phase I/II clinical trials for cancer treatment [99].
Bombesin
Bombesin (BN) receptor (BNR), also known as gastrin-releasing peptide (GRP) receptor, belongs to the GPCR superfamily and has been found to be overexpressed in lung cancer, prostate cancer, breast cancer, and pancreatic cancer [100]. In preclinical studies, some nonradioactively labeled ligand-drug complexes constructed by the combination of BN with camptothecin (CPT), doxorubicin (DOX), PTX, and other chemotherapeutic drugs have successfully improved the selectivity and efficacy of these drugs [101]. A nanostructured lipid carrier (NLC) with DOX and DNA-loaded BN-coupled polyethylene glycol (PEG) stearate (BN-PEG-SA) was synthesized and found to serve as a better carrier for improved cellular targeting and nuclear targeting, and its nanodrug may be a promising active targeting drug/gene therapy system for lung cancer treatment [102].
N-acetyl-d-glucosamine
Glucose can be used as a targeted molecule in the drug delivery system to promote drug transport and endocytosis [103]. Glucose transporters (GLUT), such as GLUT-1, are overexpressed in tumors of the brain, colon, liver, and lung [104]. GLUTs targeting various tumors have been successfully used for positron emission tomography, magnetic resonance contrast imaging, and gene targeting. As a glucose receptor targeting ligand, N-acetyl-d-glucosamine (GlcNAc) has good water solubility, which is helpful for enhancing the solubility and internalization of NDDSs. A synthetic polymeric drug conjugate, PEG-DOX conjugate, coupled to GlcNAc as a tumor tissue-targeting ligand, showed significantly enhanced cytotoxicity and stronger internalization and retention in cancer cells [105].
Hyaluronic acid
Hyaluronic acid (HA) is a widely distributed extracellular matrix polysaccharide with biocompatible and biodegradable properties that belongs to the glycosaminoglycan family [106]. The linear structure of HA is composed of alternating glucuronic acid (GlcA) and GlcNAc, forming a disaccharide β-d-GlcA-(1→3)-β-d-GlcNAc-(1→4) repeat sequence [107, 108]. HA is the ligand of the overexpressed CD44 receptor in NSCLC cell lines [109]. Mattheolabakis et al. [110] demonstrated that HA-modified polymer nanoparticles (PNPs) can improve the accumulation of DOX in tumor cells and improve antitumor efficiency through their involvement in the regulation of inflammation, tumor development, and healing processes [110]. Hsiao et al. [111] discussed the potential of HA-modified nanoparticles in improving apoptosis, cytotoxicity, and anti-proliferation of A549 lung cancer cells.
Trophoblast cell-surface antigen 2 antibody
Human trophoblast cell-surface antigen 2 (Trop2) is a transmembrane glycoprotein with an extracellular EGF-like and thyroglobulin 1 repeat domain [112], which is overexpressed in various solid tumors, including NSCLC [113]. High expression of Trop2 is associated with the growth and proliferation of cancer cells and the low survival rate of patients. Trop2 can recognize specific ligands, such as insulin like growth factor-1 (IGF-1), cyclin D1 [114], and protein kinase C (PKC) [115], participate in intracellular signal transduction pathways, and regulate the cell cycle [116]. Trop2 can directly bind to IGF-1 to weaken the activation of AKT/b-catenin and ERK mediated by IGF-1R signaling. Currently, various novel antibody-drug conjugates have been developed, such as sacituzumab govitecan [117] and datopotamab deruxtecan [118], and the coupling of human anti-Trop2 antibodies with cytotoxic drugs will promote the development of tumor therapeutic targets.
NDDSs in targeted lung cancer therapy
The current clinical approach to treating lung cancer primarily involves surgery, radiotherapy, and chemotherapy (Table S1, Table 1). However, due to the lack of effective early detection methods, most lung cancer cases are diagnosed at advanced stages involving local tumor invasion or distant metastases. In cases where surgery is not suitable, chemotherapy becomes an option [119]. Conventional chemotherapy in NSCLC has limitations, including lower efficacy, higher toxicity, increased recurrence rates, and lower five-year survival rates [120]. Precision treatment options for NSCLC currently include a combination of local radiotherapy, targeted therapy, and immunotherapy [121, 122]. Immunotherapy, particularly anti-PD1 antibodies such as nivolumab and pembrolizumab, in combination with platinum-based chemotherapy has shown promising results and has been approved by the FDA to improve survival rates in advanced NSCLC patients [123].
Clinically approved nanoparticle-based medicines for lung cancer therapy
Name | Active ingredient | Formulation | Clinical phase | First approval |
---|---|---|---|---|
Genexol-PM | PTX | Polymeric micelle | Approved | 2007 |
Abraxane | PTX | Albumin | Approved | 2012 |
Lipusu | PTX | Liposome | Approved | 2019 |
AGEN1181 | αCTLA-4 | Phospholipid | I/II phase | 2020 |
PEP02 | Irinotecan | Liposome | II phase | 2015 |
DM-CHOC-PEN | DNA alkylator | Lipoid (chloroethyl-cyclohexyl-nitrosourea) | I/II phase | 2021 |
NKTR-214 | IL-2 | PEG | I/II phase | 2022 |
In the field of oncology, nanotechnology has emerged as a potential solution to enhance cancer diagnosis and treatment. Nanotechnology enables targeted delivery of imaging agents and therapeutic drugs to tumor tissues, offering advantages over conventional systemic drug delivery [124]. NDDSs have garnered extensive attention for their ability to facilitate the development of more effective treatments, reduce systemic toxicity, and improve pharmacokinetics [125]. NDDSs have shown potential in the diagnosis, treatment, and prognosis of lung cancer. NDDSs offer several advantages, including an increased drug loading capacity, improved pharmacokinetic properties, passive or active targeting mechanisms for site-specific delivery, synergistic effects of multiple therapeutic agents, minimized drug resistance, and controlled release to enhance efficacy while reducing toxicity. Passive targeting of tumors is based on the EPR effect, where small nanoparticles can accumulate specifically in tumor sites through tumor vascular leakage and lymphatic drainage [126]. Nanoparticles can also interact with TAMs, enhancing their uptake within tumors [127]. Nanoparticles can be surface modified with high-affinity ligands that bind to receptors overexpressed by cancer cells, enabling targeted delivery to tumor cells and metastatic lesions. Stimulus-responsive crosslinked nanomedicines in the field of cancer treatment and have shown advances in circumventing the drawbacks of conventional drug delivery systems [128] and are classified into three categories based on crosslinking strategies, including built-in, on-surface, and interparticle crosslinking nanomedicines. Due to the stimulus-responsive crosslinkages, stimulus-responsive nanomedicines are capable of maintaining robust stability during systemic circulation. They also respond to particular tumoral conditions to induce a series of dynamic changes, such as changes in size, surface charge, targeting moieties, integrity, and imaging signals. These characteristics allow them to efficiently overcome different biological barriers and substantially improve drug delivery efficiency, tumor-targeting ability, and imaging sensitivities. In addition, micro/nanorobots are propelled by chemical reactions, physical fields, and biological systems and can be manipulated by chemotaxis, remote magnetic guidance, and light [129]. Moreover, self-adaptive nanomaterials, which respond to signal changes emitted from the tumor site, might realize spatiotemporally and quantitatively specific release of drugs. Self-adaptive nanomaterials exhibit self-regulation and self-feedback capabilities, and their properties, such as charge, size, and shape, undergo on-demand transformation in response to specific stimuli. Compared to conventional nanomaterials, self-adaptive nanomaterials successfully decrease the frequency of drug release within normal tissues and maintain drug concentrations in tumor cells for a more extended period, thus promoting rational clinical drug application [130]. Clinical use of nanoparticles has demonstrated improved efficacy and reduced adverse effects through adjustment of the systemic biodistribution of drugs, allowing higher doses of therapeutic agents to reach tumor sites. For example, the FDA-approved albumin-bound PTX drug Abraxane showed superior overall response rates and fewer adverse events than generic PTX in NSCLC patients [131].
Research progress in nanomaterials for lung cancer treatment involves organic nanomaterials [liposomes, polymers, covalent organic framework (COF)], inorganic nanomaterials [gold, paramagnetic iron oxide, silica, carbon quantum dots, metal organic framework (MOF)], and biomimetic nanomaterials (albumin, biofilm materials, Figure 2). The size and composition of nanoparticles significantly influence their bioavailability in vivo, allowing them to pass through specific barriers while limiting uptake in healthy tissues [132, 133]. In summary, nanotechnology, particularly NDDSs, holds great promise for improving lung cancer treatment outcomes. Ongoing research and development of various nanomaterials contribute to advancing the field of lung cancer therapy.
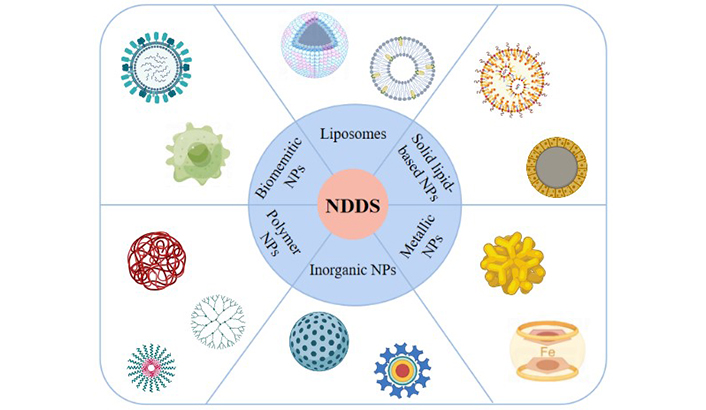
Nano-based drug delivery system for lung cancer therapy. Created by BioRender. NPs: nanoparticles
Liposomes
Liposomes are spherical bilayer vesicles formed by the self-assembly of cholesterol and phospholipids [134] and are structurally similar to biological membranes and capable of efficiently integrating hydrophobic and hydrophilic drugs into hydrophobic cavities and lipid bilayers. Liposomes as drug carriers have the following advantages: (i) nontoxic, safe, and biocompatible carrier materials; (ii) high drug loading efficiency, good stability, and a prolonged drug half-life; (iii) increased drug uptake by tumor cells; (iv) easy surface modification, which can leverage the difference between cancerous and healthy tissues to increase the residence of liposomes at the target site, enhance targeting and reduce the cytotoxicity of drugs [135]; and (v) stimulus-responsive control of drug release that can be achieved by different mechanisms, such as pH and enzymes, depending on the physicochemical properties of the drug to increase efficacy and reduce toxicity. Since cationic liposomes can self-assemble with nucleic acids, many studies have used cationic liposomes to deliver genes to the lung [136]. The main materials used in the preparation of liposomes are soybean phosphatidylcholine, cholesterol, mannitol, lipoic acid-modified polypeptides, phosphoglycerate mutase, and citraconic anhydride-grafted poly-L-lysine, among others. Zhang et al. [137] developed a liposomal curcumin dry powder inhaler for the treatment of primary lung cancer. The uptake of curcumin liposomes by A549 cells was markedly greater and faster than that of free curcumin. Zhang et al. [138] designed a tumor cell membrane-liposome hybrid bionanoparticle surface modified with a peptide targeting MMP-9 with a negatively charged citric anhydride-grafted poly-L-lysine intermediate layer for pH-triggered charge reversal and codelivery of phosphoglycerate mutase 1 small interfering RNA (PGAM1 siRNA) and docetaxel (DTX) to achieve synergistic drug effects of metabolic therapy and chemotherapy. Hu et al. [139] fused tumor-derived nanovesicles with liposomes with a homologous targeting capability and bionic hybrid nanovesicles loaded with DOX [DOX-loaded biomimetic hybrid nanovesicles (DOX@LINV)], which improved the immunosuppressive TME by infiltrating effector immune cells.
Drug resistance affects the prognosis and survival of lung cancer patients. The mechanisms associated with MDR in lung cancer include drug inactivation, DNA repair, elevated drug release from transporters such as ATP-binding cassette (ABC) transporter proteins and apoptosis defects [140]. The main protein transporter involved in pump resistance is the MDR-associated protein [141], an efflux pump that reduces intracellular drug levels. Off-pump resistance is mainly due to defective apoptosis, and the Bcl-2 protein is a major player in this mechanism [142, 143]. A dual liposome system consisting of adriamycin and siRNA blocked the MDR of Bcl-2 [144]. Delivery of DOX, Bcl-2, and MDR-associated protein 1 via liposomes markedly reduced MDR by stimulating caspase-dependent apoptotic pathways [145]. Li et al. [146] delivered oxygen and erlotinib through aptamer-modified liposome complexes to reverse hypoxia-induced drug resistance in lung cancer.
Solid lipid-based nanoparticles
The use of solid lipid-based nanoparticles (SLBNs) in drug delivery has been studied extensively. The two types of SLBNs are solid lipid nanoparticles (SLNs) and NLCs [147]. SLNs can be understood as nanoemulsions in which the liquid lipid nuclei of the droplets are replaced by solid lipid nuclei, such as triglycerides, glycerides, fatty acids, palmitates, and steroids. They are usually prepared using high-pressure homogenization or microemulsification techniques [148] and have colloidal properties, a suitable size, a high surface area-to-volume ratio, good surface properties, physical stability for controlled drug release and easy diffusion, improved stability in the presence of light, humidity, and chemically unstable drugs, and good biocompatibility. SLNs are mainly composed of the materials squalene, soybean phosphatidylcholine, Tween-80, 1,2-dioleoyloxy-3-trimethylammonium-propane (DOTAP), distearoylphosphatidylethanolamine-PEG2000 (DSPE-PEG2000), stearyl amine, monostearin, and poly-lactide-co-glycolide. More active component spaces of NLCs can be obtained by mixing solid and liquid lipids in different proportions to form different lipid matrices [149]. NLCs show better gas atomization characteristics, better accumulation in the lung, good stability, and biological activity integrity [150]. NLC-based drug delivery systems clinical applications are further advanced by various excipients, such as solid lipids, liquid-phase lipids, and surfactants [151], and the inclusion of various cationic components, target/ligand linkers, and bioactive genetic material [152].
The combination of EGFR TKIs and other chemotherapy drugs in NSCLC is a feasible strategy to overcome EGFR TKI resistance [153]. Garbuzenko et al. [154] developed NLC systems targeting luteinizing hormone-releasing hormone (LHRH) in prepared NSCLC cells to form inhaled LHRH-NLC-siRNA-PTX nanoparticles and tested them using human lung cancer cells with different sensitivities to gefitinib (EGFR inhibitor) and in situ NSCLC mouse models. Yang et al. [155] prepared a lung inhalation microsphere system for SLNs based on stearic acid-loaded afatinib (AFT) spheres [porous microspheres (pMS)] to form AFT-SLN-PTX-pMS nanoparticles. Due to the large surface area of pMS and the high initial burst release that facilitates the rapid release of PTX, this pulmonary inhalation microsphere system has the advantages of long-lasting performance, a high lung deposition rate, rapid release of PTX, sustained release of AFT, and the synergistic effects of AFT and PTX and can be used for the treatment of EGFR TKI-resistant NSCLC. Soni et al. [156] investigated the construction of gemcitabine (GEM)-loaded mannosylated SLNs with superior in vivo pharmacokinetics for effective intracellular delivery to lung cancer cells with the help of SLNs with slow controlled release and a high drug loading rate to improve drug stability, reduce toxicity, enhance efficacy, and improve pharmacokinetics. Moreover, targeted mannose-based SLNs (M-SLNs) were taken up by lung cancer cells through mannose receptor-mediated endocytosis, enhancing the cytotoxic effect on tumor cells and reducing cytotoxicity to normal cells.
Metallic and inorganic nanoparticles
Metallic and inorganic nanoparticle as drug delivery carriers have the advantages of a large surface area-to-volume ratio, surface modification of organic materials, being biological molecules, and no immunogenicity. A variety of metal and inorganic nanoparticles have been used for the treatment of NSCLC, including magnetic nanoparticles (MNPs), MOFs, gold nanoparticle, graphene oxide (GO) nanoparticle, quantum dots, plasma, mesoporous silica (MS) nanoparticles (MSNs), and black phosphorus (BP), among others. Their physical and chemical properties are related to size, shape, and composition. The diverse structures and characteristics of nanoparticles provide scaffolds for drug treatment and imaging [157], enhanced stability and bioavailability of anticancer drugs, and sustained control of drug release rates, enabling efficient and targeted treatment of cancer.
Metallic nanoparticle as drug carriers have the advantages of a high drug-carrying capacity, functional modifiability, and nonimmunogenicity. Such nanoparticle include Au nanoparticle, Cu nanoparticle, MnO2 nanoparticles, Fe3O4 MNPs, and MOFs, which are used as drug delivery carriers for lung cancer therapy. Particle-based pulmonary drug delivery has considerable potential to provide inhalable therapeutics for local or systemic applications. Designing particles with enhanced aerodynamic properties can improve lung distribution and deposition, thereby enhancing the efficacy of capsule-based inhaled drugs. As nanocarriers for the inhalation of small-molecule drugs and macromolecular drugs, metallic phenol capsules can increase the thickness of the capsule shell by repeatedly depositing thin films on the template, thereby increasing the aerodynamic diameter and accurately controlling the deposition of the capsule shell in a human lung model [158].
An important therapeutic approach based on metal materials is magnetic nanotherapy, a noninvasive method of tumor ablation based on MNPs acting on their own or with anticancer drugs and external magnetic fields, where drug-laden nanoparticles can be guided into the circulatory system and targeted tissues under the action of an alternating magnetic field [159, 160]. Iron oxide nanoparticles are the most commonly used nanoparticles for MNP therapy due to their degradability, biocompatibility, and superparamagnetic effect [161]. Iron ions can catalyze the generation of free radicals from hydrogen peroxide through the Fenton-type reaction, which can damage mitochondria, lipids, proteins, DNA, and other structures in tumor cells and induce apoptosis [162, 163]. Tseng et al. [164] showed that recombinant adeno-associated virus serotype 2 (AAV2) chemically conjugated with iron oxide nanoparticles (approximately 5 nm) has a remarkable ability to be remotely guided under a magnetic field. Transduction is achieved with microscale precision. Furthermore, a gene for the production of the photosensitive protein KillerRed was introduced into the AAV2 genome to enable photodynamic therapy (PDT) or light-triggered virotherapy. In vivo animal experiments revealed that magnetic guidance of “ironized” AAV2-KillerRed injected through the tail vein in conjunction with PDT significantly decreased tumor growth via apoptosis. Sadhukha et al. [165] investigated the synthesis of inhalable superparamagnetic iron oxide (SPIO) nanoparticle (SPIONs) targeting EGFR, and the results confirmed that magnetic thermotherapy using SPIO-targeted nanoparticles significantly inhibited tumor growth in vivo and that magnetic thermotherapy has strong potential as a treatment modality for NSCLC. Huang et al. [166] combined porous iron oxide nanoagents (PION)-mediated promoting photothermal therapy (PTT) with CRYBG3 long noncoding RNA (lncRNA)-mediated gene therapy, where PIONs were used as a magnetic nanoagent for magnetic resonance imaging (MRI) and photoacoustic imaging (PAI), and a high cancer cell killing effect was observed in vitro and in vivo.
Functional modification of nanocarriers can enable integration of multiple functions into NDDSs to achieve targeted drug delivery, diagnostic imaging, and combined therapy. Ma et al. [167] used an integrated therapeutic and diagnostic targeting nanoplatform for lung cancer in situ spinal metastases for the first time (Figure 3). Au@MOF was coated with MSNs and connected with the photosensitizer indocyanine green (ICG) to alter the modified targeting peptide dYNH on Au@MOF@MS-ICG. The dYNH peptide was combined with the transmembrane receptor erythropoietin, which produces human hepatocellular A2 (EphA2) [168], and the outer layer was modified with polyacrylic acid (PAA) to enable pH-sensitive codelivery of cisplatin and the alpha-selective PI3K inhibitor BYL719@Au@MOF@MS-ICG, representing an integrated therapeutic nanoplatform combining dual drugs, strong targeting, and photothermal effects. In addition, imaging modalities are expected to serve as an efficient and safe smart drug delivery system for the treatment of in situ metastases.
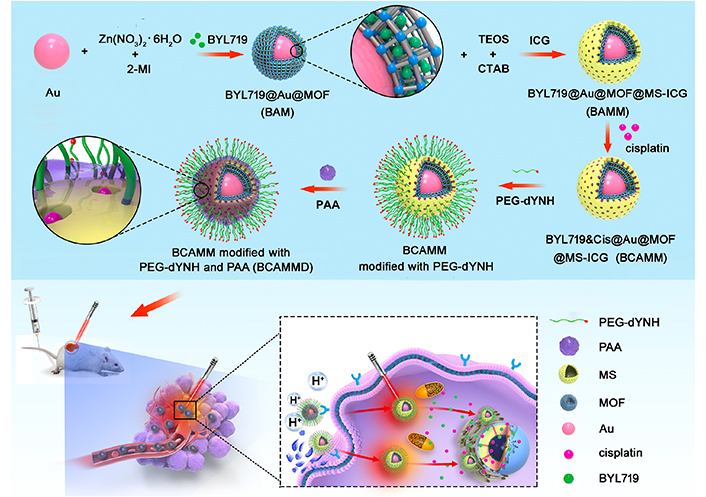
Synthesis of BCAMMD modified bivalve nanoparticles (BCAMM) loaded with BYL719-cisplatin. BCAMM: BYL719&cisplatin@Au@MOF@MS-ICG; BCAMMD: dYNH-targeted peptide; Cis: cisplatin; CTAB: hexadecyltrimethylammonium chloride solution; MI: methylimidazole; TEOS: tetraethyl orthosilicate
Note. Reprinted with permission from “Rationally integrating peptide-induced targeting and multimodal therapies in a dual-shell theranostic platform for orthotopic metastatic spinal tumors” by Ma Y, Chen L, Li X, Hu A, Wang H, Zhou H, et al. Biomaterials. 2021;275:120917 (https://linkinghub.elsevier.com/retrieve/pii/S0142961221002738). © Elsevier 2021.
Inorganic nonmetallic nanoparticles have unique physicochemical properties and biological effects; for instance, GO nanoparticle can effectively load and deliver antigens, show the potential to activate the immune system, have easily modified surfaces, and are widely used as carriers and immune adjuvants [169]. BP nanoparticle are a new type of phosphorus-source nanoparticle, and BP quantum dots (BPQDs) and BP hydrogels (BPHs) are both common forms BP. Due to their high photothermal conversion efficiency, excellent PTT and PDT and good biocompatibility, BP-based drug delivery systems have received attention and are widely used [170].
Quantum dot-based fluorescence strategies can improve sensing performance and enhance sensitivity to targets due to their optical and photophysical properties and adjustable emission range [171, 172]. Moreover, integrating organic dyes and quantum dots into peptide substrates provides a well-controlled and scalable strategy for protease-sensing fluorescence resonance energy transfer (FRET) systems. Wu et al. [173] constructed a nitrogen-rich carbon dot (NCD)-mediated DNA nanostructure self-assembly strategy. Due to the excellent photoluminescence and photodynamic properties of NCDs, NCDs can isothermally mediate DNA nanopillar self-assembly in a magnesium-free manner in large temperature and pH ranges and combine with KRAS siRNA for the treatment of KRAS mutant NSCLC. Studies have shown that the combination of NCDs and programmable DNA nanostructures is a powerful strategy for endowing DNA nanostructures with new functions, and the nanoplatform has phototherapy potential.
MSNs are one of the most widely used inorganic nanoparticles, with the advantages of large pore size, an adjustable particle size and pore diameter, a large specific surface area, a high density of silanol groups on the surface, favorable functional modification, excellent biocompatibility, and can induce reactive oxygen species generation [174], rendering MSNs ideal carriers for adsorbable drug molecules [175, 176]. Zhou et al. [177] prepared a novel injectable thermosensitive hydrogel based on MSNs and thermosensitive hydrogels for the delivery of the oral targeted drug erlotinib to achieve targeted sustained release. The erlotinib-loaded hydrogel composite (ERT@HMSNs/gel) complex showed a longer drug retention time in and around the tumor, enhancing the efficacy of NSCLC. This development also provides ideas for the design and preparation of nanodelivery systems for local antitumor therapy. Cheng et al. [178] developed a d-a-tocopheryl PEG 1000 succinate (TPGS)-functionalized polydopamine-coated MSN drug delivery systems for pH-responsive release of DOX with ideal particle size, drug loading, and drug release characteristics and long cycle advantages. Drug-resistant A549 cells were used to detect the cytotoxicity and cellular uptake of nanoparticle. NDDSs showed outstanding performance in overcoming MDR.
PNPs
PNPs are amphiphilic polymers self-assembled by hydrophobic interactions in aqueous solution to form a thermodynamically stable system. Hydrophobic small molecules are trapped within PNPs by covalent bonding or interaction with the hydrophobic core, and hydrophilic drugs can be loaded by physical action or chemical coupling to effectively carry antitumor drugs and remain stable in vivo [179]. PNPs are easy to prepare, have good biocompatibility, low toxicity, structural stability, chemical modification abilities, and multifunctional groups that can bind specific ligands or antibodies [180] to achieve the advantages of targeted drug delivery, and are widely used in drug delivery systems [181, 182], showing strong potential for chemotherapy and gene therapy. CRLX101 is a self-assembled nanoparticle containing PEG-poly(lactic acid)-encapsulated CPT that delivers CPT to cancer cells while significantly reducing systemic exposure and is currently in phase II clinical trials [183]. Tseng et al. [184] reported the exploitation of NSCLC tumor-secreted lactate in designing an acid-degradable nanoparticle containing the acyclic acetal component of oxidized HA for viral release. The virus, lactate oxidase (LOX), and hexanoamide were conjugated with aldehyde-HA through reductive amination (Figure 4) [185]. The lower pH can facilitate virus internalization into cells due to pH-sensitive proteases of the viral capsid. Site-specific delivery was demonstrated by viral transduction in the NSCLC tumor-secreted lactate microenvironment, offering an avenue for improving general or drug-resistant NSCLC treatment outcomes. The exploitation of tumor lactate production in designing a hypoxia-responsive carrier self-assembled from HA conjugated with 6-(2-nitroimidazole)hexylamine for localized release of recombinant AAV2 has also been reported. The carrier is loaded with LOX and is permeable to small molecules such as the lactate that accumulates in a tumor [186]. Wang et al. [185] developed hierarchically responsive nanomedicine (HRNMs) self-assembled via a cyclic Arg-Gly-Asp (RGD) peptide-coupled triblock copolymer, poly(2-(hexamethyleneimino)ethyl methacrylate)-poly(oligo-(ethylene glycol) monomethyl ether methacrylate)-poly reduction-responsive CPT (PC7A-POEG-PssCPT). In circulation, RGD peptides are shielded by the POEG coating, and HRNMs achieve effective tumor aggregation through passive targeting. Upon reaching the tumor site, the acidic microenvironment induces hydrophobic to hydrophilic conversion of PC7A, and RGD peptides are exposed, enhancing tumor retention and intracellularization. Furthermore, HRNMs show effective tumor targeting, potent antitumor effects, and reduced systemic toxicity. Such HRNMs are expected to be used to enhance chemotherapeutic delivery. Zhong et al. [187] designed a series of biodegradable PEG, guanidine-functionalised polycarbonate and polypropylene cross-ester (PEG-PGCx-PDLAy) triblock copolymers as chemotherapeutic agents that self-assemble into micellar nanoparticles against a variety of cancer cell lines, which killed cancer cells through a nonapoptotic mechanism involving significant vacuolization and subsequent membrane disruption without inducing resistance to multiple treatments at sublethal doses of the polymer. Iyer et al. [188] developed glutathione (GSH)-responsive polyurethane nanoparticles loaded with cisplatin, which showed GSH dose-dependent cisplatin release and significantly reduced in vitro survival of A549 lung cancer cells following the action of GSH-responsive polyurethane nanoparticles (GPUs) compared to that achieved with equal concentrations of free cisplatin. In vivo biodistribution studies showed that fluorescently labeled GPUs clustered in lung tumor areas and that tumor suppression was significantly improved following tail vein injection in mice. Wang et al. [189] constructed poly(lactide-co-glycolide)-PEG-FITC-poly(ethylene glycol)-amine-MSC (PLGA-PEG-FITC-MSC) nanoparticles loaded with DTX. MSCs can be used as lung-targeting drug carriers [190] and exhibit naturally high tumor affinity [191], and the MSC/nanoparticles (MSC/NP) system can effectively target drugs to lung tissue. The tumor inhibition efficiency of the MSC/nanoparticles/DTX (MSC/NP/DTX) system was similar to that of nanoparticles/DTX (NP/DTX) but at only 1/8 of the DTX dose. Chen et al. [192] prepared pH and redox dual-responsive methoxy PEG-disulfide bond-poly(beta-amino ester)-PLGA (mPEG-SS-PBAE-PLGA) nanoparticle-loaded platinum-curcumin complexes, which facilitated intracellular release and enhanced synergistic anticancer effects.
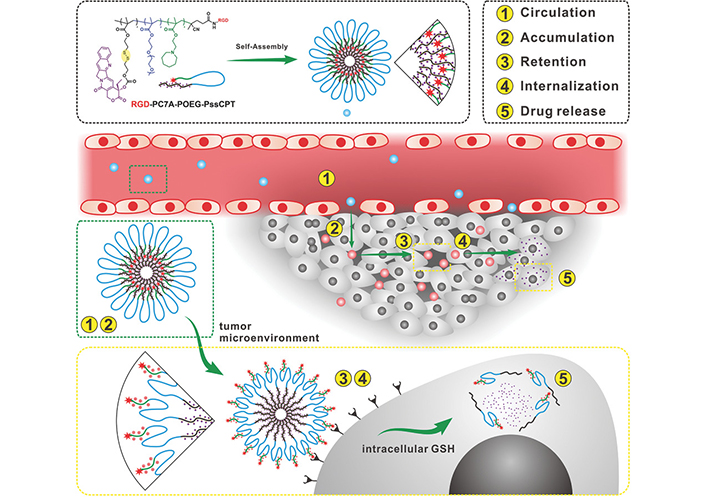
Schematic diagram of programmed administration of HRNMs. (1) HRNMs have high stability in the circulation, (2) due to their nanoparticle size and neutral POEG surface; therefore, they can effectively accumulate, within the tumor through EPR effects. (3) In the TME, the acidic pH leads to charge switching and exposure of RGD peptides by HRNMs, which enhances tumor retention and (4) intracellularization. (5) Thereafter, intracellular GSH will trigger the release of CPT from cancer chemotherapy
Note. Reprinted with permission from “Hierarchical tumor microenvironment-responsive nanomedicine for programmed delivery of chemotherapeutics” by Wang S, Yu G, Wang Z, Jacobson O, Tian R, Lin LS, et al. Adv Mater. 2018:e1803926 (https://onlinelibrary.wiley.com/doi/10.1002/adma.201803926). © WILEY-VCH Verlag GmbH & Co. KGaA, Weinheim 2018.
Many noninvasive technologies, such as near-infrared (NIR) and ultrasound therapy, have been applied to the treatment of cancer. Compared with lasers, which have a limited penetration depth, ultrasound energy can penetrate deeper tumor tissues in a safe range, which is more suitable for the treatment of lung cancer. Wang et al. [193] proposed a novel NIR light-triggered photothermal polymer containing DAP-F complexed with a reduction-sensitive amphiphilic polymer, P1, to form F-nanoparticles with photothermal effects that can encapsulate Pt(IV) prodrugs via P1 and bind F-nanoparticles to Pt-nanoparticles to construct the final nanosystem F-Pt-nanoparticles, which inhibited DNA repair, effectively overcame cisplatin resistance, and suppressed tumors (Figure 5).
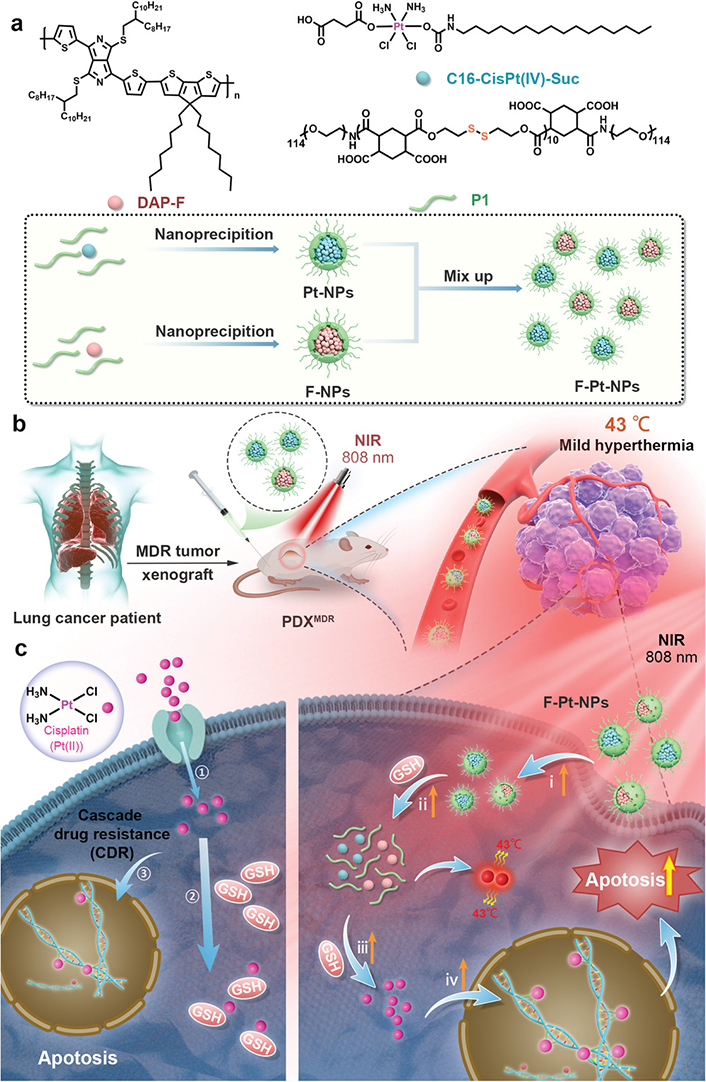
Schematic illustration of the preparation of the F-Pt-NPs and possible mechanism involved in inhibition of cisplatin resistance under NIR laser irradiation. PDX: patient-derived xenograft
Note. Reprinted with permission from “A systematic strategy of combinational blow for overcoming cascade drug resistance via NIR-light-triggered hyperthermia” by Wang L, Yu Y, Wei D, Zhang L, Zhang X, Zhang G, et al. Adv Mater. 2021;33:e2100599 (https://onlinelibrary.wiley.com/doi/10.1002/adma.202100599). © Wiley-VCH GmbH 2021.
Biomimetic nanoparticles
Biomimetic nanoparticles have the morphology, surface properties, and size of natural constituent structures (e.g., exosomes) that evade clearance by the immune system, an enhanced targeting ability to deliver drugs to target cells or tissues, good biocompatibility, improved therapeutic efficiency, and reduced toxic side effects and are widely studied as drug delivery systems. With hybridization, nucleic acid nanoassemblies, including DNA and RNA nanostructures, can be applied to enhance diagnosis and therapeutic effects [194]. Kuerban et al. [195] obtained outer-membrane vesicles (OMVs) from attenuated Klebsiella pneumonia and prepared DOX-loaded OMVs, which were efficiently transported into A549 cells and presented substantial tumor growth inhibition with favorable tolerability and pharmacokinetic profiles. The appropriate immunogenicity of OMVs enables the recruitment of macrophages to the TME, which might synergize with DOX in vivo. Li et al. [196] found that inhibition of exosome secretion may be an effective strategy to overcome the antagonistic effects of TKIs and chemotherapeutic agents. Zhong et al. [197] constructed three different DNA nanostructures and found that DNA nanostructures could significantly enhance the intracellularization of platinum drugs, thereby increasing their anticancer activity not only against conventional A549 cells but also more importantly against cisplatin-resistant (CisR) cancer cells (A549 CisR), thus effectively inhibiting tumor growth, suggesting that DNA nanostructures are effective carriers for platinum precursor drug delivery to counter chemotherapy resistance.
Proteins, such as albumin [198] and high-density lipoproteins (HDLs) [199], and silk fibroin nanoparticles (SFNPs) [200] have been used to treat NSCLC, and their amino and carboxylic acid fractions can be chemically modified to actively target cancer cells. Among all types of polypeptide nanoparticles, polycystine 2 (PCys2)-based nanoparticles have drawn increasing attention due to their unique properties. On the one hand, uniform nanogels can be easily obtained through the crosslinking of two active centers during polymerization without an additional self-assembly step. On the other hand, the Cys2-based nanoparticles always showed reduction responsiveness owing to the inherent disulfide bond. With the development of advanced diagnostic and therapeutic technologies, multifunctional PCys2-based nanoparticles were achieved via rational construction of the polymer structure [201]. Elgohary et al. [202] developed inhalable human serum albumin (HSA) nanocomposites for the combined delivery of etoposide (ETP) and berberine (BER) to lung tumors, which reduced toxicity, prevented drug resistance, and enhanced cytotoxicity and internalization in A549 lung cancer cells. Cell and cell membrane-derived nanobiocarriers are being developed to design new drug delivery strategies for the treatment of various malignant tumors, including lung cancer. At present, the sources of this biological nanocarrier mainly include red blood cells, white blood cells, platelets, MSCs, cancer cells, and exosomes. Cell-based nanobiocarriers have good biocompatibility, multimolecular and intrinsic targeting abilities, a long cycle ability, and good host biointegration and can be used for the treatment of various tumors. Cell membrane-derived microparticles (MPs) are important mediators of intercellular information transfer. Guo et al. [203] investigated the therapeutic potential of tumor cell-derived MPs (TMPs) in patients with malignant pleural effusion (MPE) from lung cancer. The safety, immunogenicity, and clinical activity of TMPs-methotrexate (TMPs-MTX) were validated in a human study in 11 patients with MPE in advanced lung cancer, showing a significant reduction in tumor cells and CD163+ macrophages and stimulation of IL-2 release from CD4+ T cells and IFN-γ release from CD8+ T cells. Ye et al. [198] used neutrophil-mediated nanoparticles to promote tumor photothermal therapy by modifying Au nanorods (AuNR) with bovine serum albumin (BSA) coupled to RGD (AuNRBR) and then encapsulated them in neutrophils (AuNRBR/N). Neutrophils can efficiently cross epithelial cells and exhibit enhanced cytotoxicity against Lewis cells under in vitro laser irradiation. In addition, AuNRBR/N showed stronger targeting to tumor tissue than cell-free nanoparticles, and the enhanced tumor-homing efficiency of AuNRBR/N and the release of AuNRBR from neutrophils facilitated further deep tissue dissemination, contributing to tumor growth inhibition and improved survival in PTT.
Conclusions
Patients with lung cancer often face low overall survival, progression-free survival, and quality of life. Current interventions for lung cancer primarily target advanced stages of the disease characterized by signs of tumor metastasis and recurrence, which are influenced by genetic mutations, drug resistance, and epithelial-mesenchymal transition (EMT) processes [204]. While new therapies combined with traditional drugs have shown some clinical success, NDDSs have become increasingly important in clinical trials and applications. NDDSs offer the potential to enhance treatment efficacy and reduce side effects by modulating the biodistribution and pharmacokinetics of drugs. However, despite promising results, further research is necessary to develop more effective nanoplatforms. Currently, passive targeting based on the EPR effect is a common approach for tumor uptake of nanobased agents [205]. However, the EPR effect is typically less prominent in humans than in animal models [206]. To address the limited tumor uptake, nanoparticles can be designed to actively target tumor tissue-specific overexpressed proteins using a combination of active and passive targeting strategies, facilitating receptor-mediated endocytosis. This active targeting strategy aims to simultaneously target primary and metastatic tumor cells [207], as metastatic cells may not exhibit strong EPR effects in the early stages.
However, many remaining challenges require considerable research. While NDDSs have shown promise in overcoming drug resistance in lung cancer treatment, the exact mechanisms by which these systems can effectively deliver drugs to tumor cells and bypass drug resistance mechanisms are still not well understood. Further research is needed to elucidate the specific interactions between nanoparticle-based drug carriers and tumor cells regarding drug resistance. In addition, although many different types of nanoparticles have been used for lung cancer therapy, a consensus on the optimal characteristics of nanoparticle design for effective drug delivery is still lacking. Research is needed to determine the influence of nanoparticle size, shape, surface properties, and targeting ligands on the efficiency of drug delivery to lung tumor cells. Understanding the pharmacokinetics, biodistribution, and clearance of NDDSs is crucial for their successful translation into clinical use. More studies are needed to investigate the fate of nanoparticles in the lung, including their distribution within tumor tissues, metabolism, and excretion pathways. While NDDSs hold great promise, their potential toxicity and safety concerns need to be thoroughly investigated. Systemic administration of nanoparticles for lung cancer therapy may lead to off-target effects and accumulation in healthy tissues, which could have adverse toxicological effects. Further research is needed to assess the safety profiles of different nanoparticle formulations and develop strategies to minimize potential toxicity. Preclinical studies have explored active targeting strategies using EGFR-targeting peptides [165, 208], HA [209], folic acid [210, 211], Tf, and other ligands in lung cancer models. Despite significant advancements in the preclinical development of NDDSs for lung cancer therapy, very few have been successfully translated to clinical use. However, clinical trials involving these active targeting agents in lung cancer patients are still underway. Considering the heterogeneity of tumors, employing multiple ligands targeting different cell surface receptors is preferable [212]. Alternatively, specific targeted agents can be designed based on the individualized driver mutation characteristics of different subtypes of lung cancer considering the TME and controlling drug release to minimize off-target toxicity and enhance precision therapeutic effects. Research gaps exist in terms of the scalability, manufacturing reproducibility, and clinical feasibility of these systems. Further studies are needed to address the challenges related to large-scale production and clinical translation of effective nanodrug delivery platforms for lung cancer treatment. Currently, clinical treatment with nanomedicines in lung cancer patients shows promise. However, further improvements in their structures and the combination of multiple NDDS approaches are necessary to provide multidrug treatment options and achieve optimal NDDSs with superior functional and structural properties, high reproducibility, simple preparation methods, and low costs.
Abbreviations
AAV2: | adeno-associated virus serotype 2 |
AFT: | afatinib |
AKT: | protein kinase B |
ALK: | anaplastic lymphoma kinase |
Bcl-2: | B cell lymphoma 2 |
BN: | bombesin |
BP: | black phosphorus |
BRAF: | v-raf murine sarcoma viral oncogene homolog B1 |
CPT: | camptothecin |
CTLA4: | cytotoxic T lymphocyte associated protein 4 |
DOX: | doxorubicin |
DTX: | docetaxel |
EGF: | epidermal growth factor |
EGFR: | epidermal growth factor receptor |
EPR: | enhanced permeability and retention |
ErbB: | Erb-B2 receptor tyrosine kinase 2 |
ERK: | extracellular signal-regulated kinase |
FDA: | U.S. Food and Drug Administration |
FR: | folate receptor |
GlcNAc: | N-acetyl-d-glucosamine |
GLUT: | glucose transporter |
GPCR: | G-protein-coupled receptor |
GSH: | glutathione |
GTP: | guanosine triphosphate |
HA: | hyaluronic acid |
HER: | human epidermal growth factor receptor |
HGF: | hepatocyte growth factor |
HGFR: | hepatocyte growth factor receptor |
HRNMs: | hierarchically responsive nanomedicine |
ICG: | indocyanine green |
IGF-1: | insulin like growth factor-1 |
JAK: | Janus kinase |
KRAS: | Kirsten rat sarcoma virus oncogene homolog |
MDR: | multidrug resistance |
MEK1: | mitogen-activated protein kinase kinase 1 |
MET: | mesenchymal-epithelial transition factor |
MNPs: | magnetic nanoparticles |
MOF: | metal organic framework |
MS: | mesoporous silica |
MSCs: | mesenchymal stem cells |
MSNs: | mesoporous silica nanoparticles |
NCD: | nitrogen-rich carbon dot |
NDDS: | nanodrug delivery system |
NIR: | near-infrared |
NK: | natural killer |
NLC: | nanostructured lipid carrier |
NSCLC: | non-small cell lung cancer |
OMVs: | outer-membrane vesicles |
PAA: | polyacrylic acid |
PC7A: | peptide-coupled triblock copolymer, poly(2-(hexamethyleneimino)ethyl methacrylate) |
PD-L1: | programmed cell death ligand 1 |
PDT: | photodynamic therapy |
PEG: | polyethylene glycol |
PI3K: | phosphatidylinositol 3-kinase |
pMS: | porous microspheres |
PNPs: | polymer nanoparticles |
POEG: | poly(oligo-(ethylene glycol) monomethyl ether methacrylate) |
PTT: | promoting photothermal therapy |
PTX: | paclitaxel |
RAF: | rapidly accelerated fibrosarcoma |
RAS: | rat sarcoma |
RGD: | Arg-Gly-Asp |
ROS1: | ROS proto-oncogene 1-receptor tyrosine kinase |
SCLC: | small cell lung cancer |
siRNA: | small interfering RNA |
SLNs: | solid lipid nanoparticles |
STAT: | signal transducer and activator of transcription |
TAMs: | tumor-associated macrophages |
Tf: | transferrin |
TKIs: | tyrosine kinase inhibitors |
TKs: | tyrosine kinases |
TME: | tumor microenvironment |
TRAIL: | tumor necrosis factor-related apoptosis-inducing ligand |
Trop2: | trophoblast cell-surface antigen 2 |
VEGF: | vascular endothelial growth factor |
VEGFR: | vascular endothelial growth factor receptor |
β2-ARs: | β2-adrenergic receptors |
Supplementary materials
The supplementary material for this article is available at: https://www.explorationpub.com/uploads/Article/file/1001221_sup_1.pdf
Declarations
Author contributions
SZ: Conceptualization, Writing—original draft. XL and YL: Writing—review & editing. HL and ZZ: Supervision.
Conflicts of interest
The authors declare no conflicts of interest.
Ethical approval
Not applicable.
Consent to participate
Not applicable.
Consent to publication
Not applicable.
Availability of data and materials
Not applicable.
Funding
This work was supported by the National Natural Science Foundation of China [82003680, 82111530241], Shandong Provincial Natural Science Foundation [ZR2020QH350, ZR2021QH024, ZR2023YQ065], Open Projects Fund of NMPA Key Laboratory for Technology Research and Evaluation of Drug Products [No. 2022TREDP03], and the Chinese “post-doctoral international exchange program”. The funders had no role in study design, data collection and analysis, decision to publish, or preparation of the manuscript.
Copyright
© The Author(s) 2024.