Abstract
Aim:
Osteoporosis (OP) is caused by imbalanced bone remodelling homeostasis. It is highly prevalent, especially in post-menopausal women, resulting in high risk of fracture and morbidity. Mesenchymal stromal cells (MSCs) are osteoblast progenitors, and orchestrate the function of surrounding cells including osteoblasts. Understanding MSC phenotype and function is therefore critical in discerning the aetiology of OP and developing superior therapies. Currently, adequate long-term therapeutic strategies are not available.
Methods:
Bioinformatic analysis of ribonucleic acid sequencing (RNA-seq) data revealed differential expression of genes primarily related to osteogenic differentiation and proliferation, followed by confirmatory in vitro analysis.
Results:
This study identified novel and previously proposed targets for therapeutic intervention in OP. Functional assessment demonstrated reduced MSC number and osteogenic capacity associated with OP. Proliferation was not affected but OP was unexpectedly associated with a reduction in MSC adipogenic differentiation capacity, correlating with donor age.
Conclusions:
These data indicate specific targets for further studies of future treatments for OP, including the assessment of modified MSCs as therapeutics. Advances in this area may contribute to reducing fracture-associated morbidity and mortality, and improving quality of life for the 200 million people living with OP globally.
Keywords
Osteoporosis, mesenchymal stromal cell, mesenchymal stem cell, bone marrow stromal cells, osteogenesis, differentiationIntroduction
Osteoporosis (OP) is a highly prevalent bone weakening disease. An increased risk of bone fracture, including associated loss of independence and quality of life, is the primary clinical concern. Rates of disease onset increase with age for both genders but more drastically in women after menopause [1]. On a physiological level, loss of bone density is the hallmark of OP, whilst on a molecular level it is an imbalance between osteoclasts which resorb bone, and mesenchymal stromal cell (MSC)-derived osteoblast synthesis of new bone [2, 3]. Osteoblasts deposit proteins and minerals before becoming ensconced in the extracellular matrix whereupon they undergo apoptosis or differentiation to osteocytes [4]. Bone formation and bone resorption both increase in response to the drastic dip in estradiol levels in women during menopause, however, while the former rises by on average 45%, the latter responds with a much greater increase to almost double pre-menopausal bone resorption levels [2].
Alongside this relative drop in bone formation is a more gradual age-associated increase in the adiposity of the bone marrow compartment [5]. As osteoblasts and adipocytes derive from a singular progenitor, the MSC, regulation of either will differentially impact the other depending on whether the target of the regulation is upstream or downstream of the point of fate choice. Generally, body mass index (BMI) correlates with bone mineral density (BMD) in conjunction with adipose stores in both humans and mice [6–8]. In contrast, signaling downstream of the fate choice results in preferential differentiation to either adipogenic or osteogenic lineages [9]. Several studies have linked an alteration in MSC fate-choice from osteogenesis to adipogenesis with the development of OP but independence from the confound of age remains unclear [9, 10].
Studies of bone marrow-derived human MSCs from individuals with OP have varied in their findings, with some indicating intrinsic changes such as reduced proliferative or modified differentiation capacity, others a decreased migrative ability, while others report no change in those assays and focus on changes in gene transcript or protein levels. Modifications have been reported in transcripts associated with osteogenesis and osteoclastogenesis, and to protein levels that may modify cell number [11–14]. Findings are often complicated by age differences between donor groups (age has been shown to alter MSC function in mouse and human studies [15, 16]), while others have been well age-matched but not segregated by gender, a factor with an important role in OP development [17]. Therefore, the presence of an OP-specific shift in proliferative or differentiation capacity of MSCs, as well any role in disease progression remains largely unknown. The objective of this study was a greater understanding of this process which is essential in furthering the development of therapeutics for OP considering MSCs are the source of osteoblasts and a regulator of osteoclasts. Both MSC dysfunction as a target for therapeutic repair and the use of ex vivo expanded MSCs themselves as therapeutics could have the potential to support bone formation in those people suffering from OP.
Here the functional characteristics of human bone marrow-derived MSCs from people diagnosed with OP are compared to MSCs isolated from an age- and gender-matched population in the context of transcriptomic analysis. This study identifies a reduced number of MSCs in people with OP, along with relevant transcriptomic modifications and a decrease in both osteogenic capacity and adipogenic capacity. These results inform our understanding of the aetiology of OP which provides novel opportunities for therapeutic development. Current treatments have low prescription and adherence rates partly due to high incidence of adverse events [3], therefore new interventions are needed to reduce the currently growing number of OP-related fractures.
Materials and methods
In all assays, the experimental operator was blinded to donor cohort through the procedure and its analysis. All experiments were coordinated to ensure that donors from each population cohort were evenly represented in each assay run.
Bone marrow donation
Individuals presenting in Galway University Hospitals for an elective hip replacement surgery or as a result of traumatic injury were approached for marrow donation. Following the review of a patient information leaflet and the receipt of informed consent, surgically discarded marrow was collected for research purposes. Approximately 3 mL of bone marrow was combined with 500 µL of heparin (Wockhardt, Clonmel, Ireland) at collection. The MSCs were isolated within 72 h of marrow harvest.
The donors in both cohorts were aged populations who presented with typical comorbidities. Individuals over the age of 50 admitted to Galway University Hospitals following fragility fracture were identified as having OP [18]. Control cohorts were selected from samples isolated from individuals undergoing elective hip replacement surgeries, and for each assay were age- and gender- matched to the osteoporotic donor samples to the extent possible (Table S1). Though the majority of the donors receiving hip replacement for reasons other than frailty have arthritis, arthritis is a highly prevalent condition associated with age that is also present in the cohort of donors with OP. Prioritisation was given in this study to accessing bone marrow from an age-matched population due to the numerous age-related confounding factors associated with MSCs from younger donors, and the very low prevalence in the population of an aged cohort with no musculoskeletal morbidity. Further, accessing the bone marrow of donors in this age category without prior medical need would be of ethical concern. A sample of donor charts were surveyed for bone health-relevant medications and overall only 15% of donors were found to be taking any [7% age-matched control (AMC), 22% individuals with OP]. Bone health-relevant medications recorded were calcium and vitamin D3 supplements and denosumab.
Our previous work identified that as a variable, gender was found to have no impact on MSC number or function in any of the assays performed [19], nevertheless considering that sufficient samples from men with OP were not possible to obtain (due to low prevalence), men were excluded from the analyses. Therefore, only women with and without OP are included in the analysis. Gender was self-identified, and no samples were received by individuals identifying as non-binary or other.
MSC isolation
MSCs were isolated from the collected marrow as previously described [19]. The collected marrow was centrifuged and the pellet resuspended in phosphate buffered saline (PBS; Gibco via Biosciences, Dun Laoghaire, Ireland, 14190094). The number of mononuclear cells (MNCs) were quantified and the cells were plated in tissue culture flasks in expansion medium [minimal essential medium α (α-MEM) with GlutaMAX™; Gibco, 32561029] supplemented with 10% fetal bovine serum (FBS; HyClone via ThermoFisher, Ballycollen, Ireland SV30160.03), 1% antibiotic antimycotic solution (Merck, Dublin, Ireland, A5955), and 1 ng/mL recombinant human fibroblast growth factor (FGF)-basic (Peprotech, London, UK, 100-18B) at 37°C with 5% CO2. Following the first passage (P0), the MSCs were lifted from the growth surface with 0.25% trypsin-ethylenediaminetetraacetic acid (EDTA; Gibco, 25200056), evenly distributed in freezing medium [10% dimethyl sulfoxide (DMSO; Sigma, Arklow, Ireland, D2650) with 90% FBS], and cryopreserved in liquid nitrogen.
Flow cytometry
Following expansion through one passage (P1), the BD Biosciences Stemflow Human MSC Analysis Kit (BD, Limerick, Ireland, 562245) was used to characterize the plastic-adherent cultures. They were profiled for expression of markers according to the International Society for Cellular Therapy criteria for the identification of human bone marrow-derived MSCs [20]. Individually conjugated CD73, CD90 and CD105 antibodies were employed to identify positive markers while a cocktail of phycoerythrin (PE)-conjugated antibodies targeting CD34, CD45, CD11b or CD14, CD19 or CD79α, and HLA-DR antibodies served to characterize expression of negative markers.
Ribonucleic acid sequencing and analysis
Total RNA was extracted from MSCs at P1 using the QIAGEN RNEasy Plus Mini Kit (Qiagen, Germany) and according to the manufacturer’s protocol. RNA quality and quantity was assessed with Nanodrop (Thermo Fisher, USA) and Agilent Bioanalyser 2100 with RNA 6000 Nano LabChip Kit (Agilent Technologies, USA). Optimal RNA characteristics for sequencing were set by LC Sciences, USA [RNA amount ≥ 2 μg, 260/280 > 1.8, 260/230 > 1.0, RNA integrity number (RIN) value ≥ 7.0] who were contracted to perform sequencing, quality control, mapping and assembly of reads, and assessment of differential expression of genes and transcripts, including Kyoto Encyclopedia of Genes and Genomes (KEGG) and Gene Ontology (GO) analysis. Genes were categorised as “novel” in the context of OP when neither the gene nor protein name in combination with the search term “osteoporosis” yielded any result on PubMed.
Osteogenic differentiation
MSCs at 80% confluence were cultured in osteogenic medium consisting of 1 nmol/L dexamethasone (Merck, D4902), 100 μmol/L ascorbic acid 2-phosphate (Merck, A8960), and 10 mmol/L β-glycerophosphate (Merck, G9422) in low-glucose Dulbecco’s modified eagle medium (DMEM; Merck, D6046) with 10% FBS and 1% penicillin-streptomycin (PS; Gibco, 15140-122). Negative, undifferentiated controls were maintained in expansion medium. In both conditions, the medium was changed twice weekly for up to 17 days. Calcium deposited into the monolayer’s extracellular matrix was stained on ice with 2% Alizarin Red S (Merck, A5533) in water following fixation in 95% methanol. Images of Alizarin Red stained cultures were obtained with a 4× objective lens on an Olympus BX43 brightfield microscope fitted with HD Chrome camera (1/.8”) and 0.5× C-mount adapter. The percentage surface area stained or occupied by Alizarin Red was calculated using Fiji (version 2) [21, 22]. A colour threshold was applied to each image, establishing what delineated red staining within the image. The mask created was then transformed into a binary image and percentage surface area of stain occupying the image was quantified.
Colony forming unit assays and cumulative population doublings
Fresh marrow was plated at 3 × 105–1.5 × 106 MNC per well in 3 replicate wells of a 6-well plate with expansion medium for 8–10 days. Following expansion, colony forming unit-fibroblast (CFU-F) and colony forming unit-osteoblast (CFU-O) were assessed. CFU-Fs were identified by rinsing the cultures with PBS, followed by incubation in 10% neutral buffered formalin (Sigma, HT501128) and staining with 0.8% crystal violet (Sigma, C0775) in methanol. CFU-Os were identified following incubation with p-nitroblue tetrazolium chloride/5-bromo-4-chloro-3-indolyl phosphate (NBT/BCIP; Merck, B1911) to visualize alkaline phosphatase activity without fixation. Stained colonies were counted with an Olympus BX43 brightfield microscope. The total number of CFU-F per 100,000 MNCs was recorded following P0.
Knowing the number of CFU-Fs in a given quantity of MNCs, the total number of MSCs in the marrow isolate were calculated at P0. From P1–P5, the cumulative population doublings were quantified by recording the number of MSCs plated and harvested in each passage [19]. Knowing the number of days in a passage, the cumulative doublings over time were recorded.
Adipogenic differentiation
Adipogenic differentiation was initiated on a confluent MSC monolayer by introducing an induction medium containing 1 μmol/L dexamethasone, 10 μg/mL human recombinant insulin (Merck, 11376497001), 200 μmol/L indomethacin (Merck, I7378), and 500 μmol/L 3-isobutyl-1-methyl-xanthine (Merck, I7018) in high glucose DMEM (Gibco, D5796), with 10% FBS and 1% PS. Three days induction periods were alternated with one day maintenance phases, when the cells were cultured in α-MEM, with 10% FBS, 10 μg/mL insulin and 1% PS. Following three induction cycles, the cells were stabilized in a 7-day maintenance period before harvesting.
Oil Red O (Sigma, O0625) staining was used to visualize and quantify lipid deposition. The culture was washed in PBS, preserved in 10% neutral buffered formalin, then exposed to 0.18% Oil Red O and the residual stain removed by rinsing the culture in 60% isopropanol (Sigma, 109827). Finally, the monolayer was counterstained with Harris Modified Haematoxylin Solution (Merck, HHS16) before brightfield imaging was carried out as described above.
To quantify the amount of Oil Red O stain retained in the culture, the monolayer was washed with 99% isopropanol to extract the stain. The extract’s absorbance was measured at 490 nm using a multimode plate reader (Victor X3, Perkin Elmer, Dublin, Ireland).
Statistical analysis
Graphpad Prism (San Diego, USA) and R (version 4.0.2) with R Studio (Boston, USA) were used to analyse the resultant data from all outcome measures. Normal distribution of data was determined in Graphpad Prism by P > 0.05 on both Shapiro-Wilk and Kolmogorov-Smirnov tests. Normally distributed data were assessed by t test or Analysis of Variance (ANOVA), whilst non-normal data were assessed by Mann-Whitney. Data variance was also compared between groups using F test in Graphpad Prism, with all normally distributed datasets found to have P > 0.05.
Results
Bone marrow MSCs were successfully isolated from people with and without OP
MSCs were successfully isolated from bone marrow samples donated by people diagnosed with OP and an AMC cohort of donors unaffected by OP. Summary statistics of donor age are provided in Table S1. MSC identity was evidenced by surface marker expression, differentiative and culture phenotype (Figure 1). Flow cytometry confirmation was according to The International Society for Cellular Therapy criteria [20], i.e., ≥ 95% cells positive for CD73, CD90, and CD105, and ≤ 5% of cells positive for CD34, CD45, CD11b or CD14, CD19 or CD79α, and HLA-DR (Figure 1A–D). Confirmation was provided by functional phenotyping of the cells’ ability to form colonies, to adhere to plastic and their multilineage potential along with their appropriate fibroblastic shape (Figure 1E).
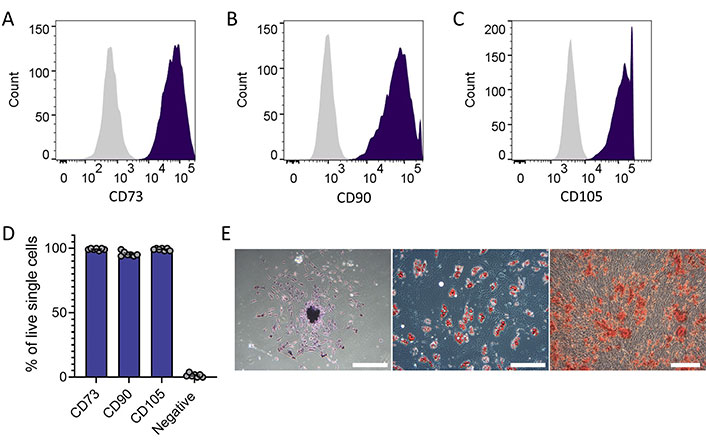
MSC identity confirmed in colony-forming adherent cells isolated from donor bone marrow (people with and without OP). (A–C) Representative histograms of flow cytometric assessment of positive MSC markers confirmed expression > 95% on all samples (n = 10), isotype control in grey; (D) summary plot of flow cytometric assessment including negative MSC markers [CD34, CD45, CD11b or CD14, CD19 or CD79α, and HLA-DR (negative cocktail)] confirmed expression < 5% on all samples (n = 10); (E) brightfield microscope images demonstrating cell adherence to plastic, colony formation (left, scale bar indicates 250 µm), and differentiation to adipocytes (middle, scale bar indicates 500 µm) and to osteocyte-like cells (right, scale bar indicates 250 µm)
MSCs from people with OP have transcriptional changes in pathways related to osteogenic regulation
RNA sequencing (RNA-seq) was performed on MSCs from both groups to identify pathways modified in the setting of OP. MSCs from people with OP exhibited altered transcriptional expression when compared with those from AMC. Only 32 genes were differentially expressed with adjusted P value (q value) ≤ 0.05, including 17 which were down-regulated, and 15 which were up-regulated. GO pathway analysis highlighted the biological processes that were significantly altered in MSCs from people with OP (Figure 2A). These pathways included skeletal system development, extracellular matrix organisation, and cell-cell interaction. We hypothesised that these gene expression changes may play a role in the capacity of MSCs to support bone homeostasis. A heatmap was generated of those differentially expressed genes which reported a consistent intragroup effect as assessed by individual analysis (Figure 2B). These 16 genes [protein kinase CGMP-dependent 1 (PRKG1), MBL associated serine protease 1 (MASP1), neurofilament light chain (NEFL), adherens junctions associated protein 1 (AJAP1), pyrimidinergic receptor P2Y6 (P2RY6), TRNA isopentenyltransferase 1 (TRIT1), family with sequence similarity 193 member B (FAM193B), ephrin B2 (EFNB2), prostaglandin D2 receptor (PTDGS), actin remodeling regulator (NHS), Rho GTPase activating protein 32 (ARHGAP32), EPH receptor B6 (EPHB6), Zinc finger protein 555 (ZNF555), telomerase associated protein 1 (TEP1), UBX domain protein 8 (UBXN8), and HAUS augmin like complex subunit 5 (HAUS5)] were then categorised according to functional involvement in processes relevant to OP; the processes of osteogenesis, proliferation, cell membrane function, cell fate choice and neuromuscular function were identified, with the majority of genes having known roles in osteogenesis (Figure 2C). Those genes which were identified by the initial bulk fold-change analysis with q ≤ 0.05 but which demonstrated P > 0.05 upon individual analysis due to high intragroup variability are presented in Figure S1.
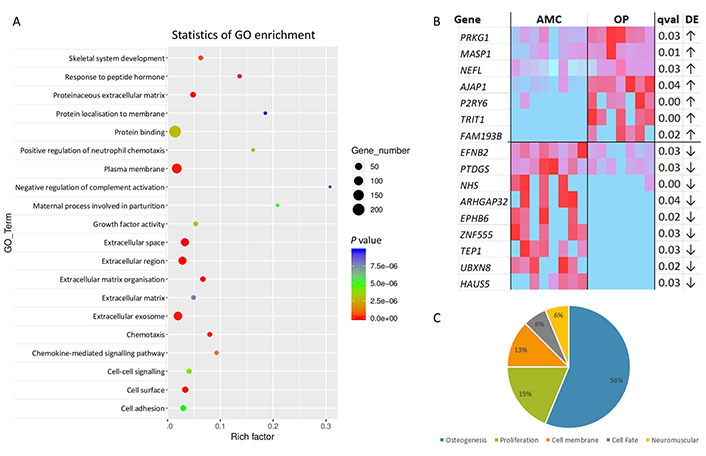
RNA-seq of MSCs from people living with OP exhibit altered expression in comparison to those from AMC donors without OP. (A) GO analysis of RNA-seq data revealing the main biological processes predicted to be affected by the observed gene expression changes between donors with OP compared to donors who do not have OP. Analysis performed by contract with LC Sciences; (B) heatmap of those genes with consistent significant differential expression between groups. Of the 32 differentially expressed genes (based on analysis of fold change q < 0.05), those with independently assessed statistical difference (P < 0.05) using t test/Mann-Whitney of fragments per kilobase of transcript per million reads mapped are presented in the heatmap; (C) pie chart summarising the proportions of genes from (B) according to known or proposed roles in pre-defined OP-relevant functions (osteogenesis, proliferation, cell membrane, cell fate, neuromuscular function). Genes with both q < 0.05 from batch analysis and P < 0.05 from individual analysis. These were classified according to pre-defined OP-relevant functions based on literature review (osteogenesis: AJAP1 [52], P2RY6 [37], PRKG1 [53], EFNB2 and EPHB6 [54], HAUS5 [55], MASP1 [56], FAM193B [57], PTDGS [58]; cellular differentiation: TRIT1 [59]; proliferation: ZNF555 [60], TEP1 [61], UBXN8 [62]; cell membrane: NHS [63], ARHGAP32 [64]; neuromuscular function: NEFL [65]). ↑: increased expression in OP; ↓: decreased expression in OP; qval: q value, i.e., corrected P value; DE: differential expression
MSCs derived from people living with OP successfully undergo osteogenesis ex vivo but at a reduced level compared to MSCs from AMC donors
Considering the significant alteration to the skeletal system development GO pathway, and the significantly different expression of genes related to osteogenesis observed, a targeted interrogation of further genes known to be involved in osteogenesis was performed. We found a statistically significant reduction in the expression of WAVE (also known as WASF1 or WASP family member 1; Mann-Whitney, P = 0.005), Fos proto-oncogene (c-fos, Mann-Whitney, P = 0.035), SPARC related modular calcium binding 1 (SMOC1; t test, P = 0.014) and natriuretic peptide receptor 3 (NPR3; t test, P = 0.009), and increased transcript levels of Reelin (Mann-Whitney, P = 0.011) and oxytocin receptor (OXTR; t test, P = 0.048) in people with OP (Figure 3A–F). There was also a trend to reduced Osterix expression (Mann-Whitney, P = 0.05) in MSCs from individuals with OP as compared to AMCs (Figure 3G). To assess the impact of the described transcript changes on the capacity of these primary cultures to differentiate to osteoblasts, MSCs from donors with or without OP were cultured in osteogenic medium and assessed for calcium deposition using Alizarin Red staining. MSCs from both donor cohorts successfully deposited calcium in the extracellular matrix in response to differentiation factors, with both groups exhibiting an increase in comparison to MSCs not exposed to differentiation medium. Modified differentiation capacity was identified by quantification of Alizarin Red staining (Figure 3H and I). This was assessed by both two-way ANOVA (P = 0.046) in Prism and binomial generalised linear model (GLM) in R (P < 0.001) and indicated a reduced osteogenic capacity associated with MSCs being derived from a person with OP. No correlation between age and osteogenesis was observed (data not shown).
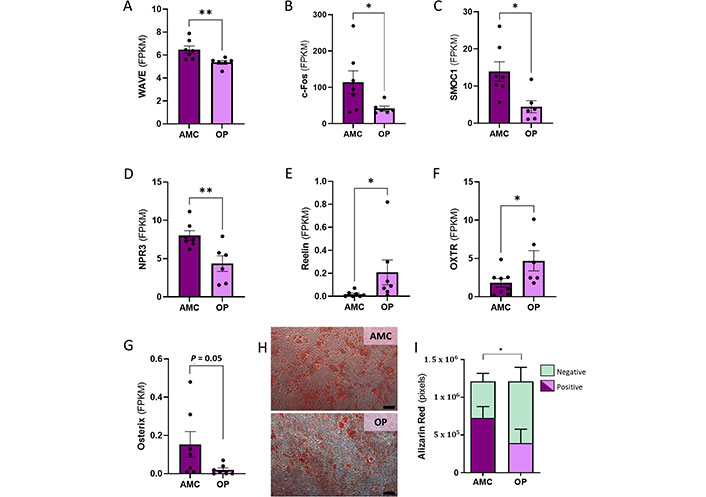
In vitro osteogenesis reduced by donor OP status coinciding with significant alteration to expression of genes related to osteogenesis. (A–G) Genes with known roles in osteogenesis and in OP development were found to have significantly altered expression in MSCs from people with OP; (H, I) a reduction was observed in the capacity of MSCs from people with OP to differentiate osteogenically in vitro when assessed by Alizarin Red staining. The scale bar indicates 250 µm. Data are represented as mean ± SEM. Data were assessed by t test where parametric and Mann-Whitney for non-parametric. SEM: standard error of the mean. * P < 0.05; ** P < 0.01
A reduced number of MSCs from people living with OP retain proliferative capacity in culture at levels similar to MSCs from control donors
Considering the observed transcriptional changes in genes related to proliferation we next assessed functional proliferative capacity. We found no difference between groups (Figure 4A and B). P0 population doublings which were calculated using MNC plating density and the known number of CFU-F/100,000 MNC were approximately equal for MSCs from both people with OP and AMC (Figure 4A). Cumulative doublings were recorded from P1 through to P5 and identified no impact of OP on proliferative capacity (Figure 4B). This adequate proliferation ex vivo was not reflected in the numbers of MSCs present in the bone marrow of people with OP as measured by counting CFU-F and CFU-O plated from fresh bone marrow samples (Figure 4C–E). Fewer MSCs were recorded from people with OP than from the AMC cohort as a proportion of the MNC population (Figure 4C). Of these MSC colonies, natively osteogenic (i.e., CFU-Os) were categorised by positive staining for alkaline phosphatase activity. CFU-O were also reduced in people with OP compared to AMC donors (Figure 4D). As the CFU-O population is a subset of the CFU-F population, we assessed the effect of OP on the proportion of CFU-O to non-osteogenic CFUs (nO-CFU, i.e., the remaining CFU-F without native osteogenic capacity). Binomial GLM analysis in R identified that the proportion of CFU-O to nO-CFU was significantly altered according to OP status of donor (using OP as the variable P = 0.014 on n = 12 OP and n = 5 AMC). There was a smaller proportion of CFU-O within the CFU-F population in samples derived from people with OP (median: 0.5/1.6, i.e., 31%) compared to AMC (median: 2.2/3.1, i.e., 71%) (Figure 4E). These data indicate that though both osteogenic and non-osteogenic MSCs are reduced in number in people with OP compared to people without OP, the osteogenic population is disproportionately reduced.
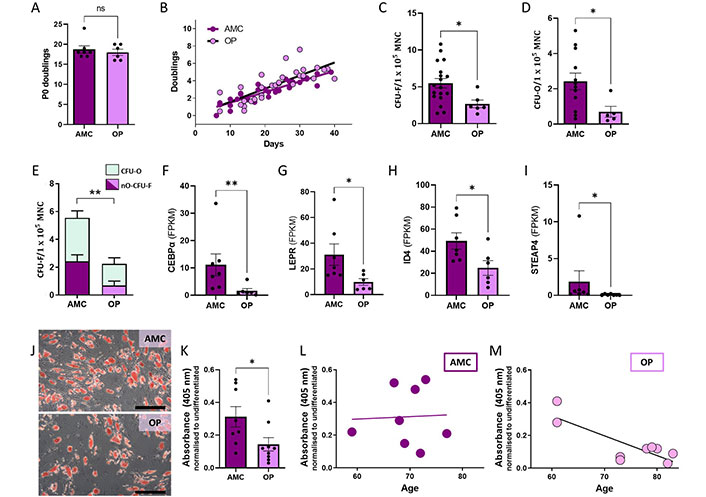
Reduced numbers and reduced adipogenic capacity of MSCs in the bone marrow of people living with OP compared to AMC. (A, B) No difference in the rate of proliferation was recorded between MSCs from donors with and without OP when analysed by t test of P0 doublings (Mann-Whiteny, P = 0.746) or by comparison of slopes using linear regression for passage 1–5 doublings (P = 0.257); (C, D) a reduced number of MSCs (CFU-F) and natively osteogenic MSCs (CFU-O) were recorded as a proportion of MNC count in samples from donors with OP compared to those from AMCs. Statistically assessed by t test (P = 0.026) and Mann-Whitney test (P = 0.022), respectively; (E) osteogenic CFU-O were preferentially depleted in OP (proportion analysis P = 0.014). nO-CFU-F (non-osteoblastic) = total CFU-F – total CFU-O; (F–I) genes with known roles in adipogenesis were found to have significantly altered expression in MSCs from people with OP; assessed by t test where parametric (ID4, P = 0.035) and Mann-Whitney for non-parametric (CEBPα P = 0.005, LEPR P = 0.022, STEAP4 P = 0.017); (J, K) reduced capacity for adipogenic differentiation, as detected by Oil Red O staining, was associated with the donor’s osteoporotic status, Mann Whitney P = 0.022. The scale bar indicates 250 µm; (L, M) there was a correlation between age and this decrease in adipogenic capacity only in donors who were diagnosed with OP (OP R2 = 0.677 P = 0.006, AMC R2 = 0.002 P = 0.917). Data are represented as mean ± SEM. ns: not significant; CEBPα: CCAAT enhancer binding protein alpha; STEAP4: STEAP4 metalloreductase; ID4: inhibitor of DNA binding 4; LEPR: leptin receptor
Age-dependent loss of adipogenesis in bone marrow MSCs from people living with OP
Previous studies have suggested preferential adipogenesis of bone marrow MSCs as a mechanism for reduced MSC osteogenesis in OP development. Since MSCs are the common progenitor for adipocytes and osteoblasts, we hypothesised that this switch in cell fate choice may be driving the observed osteogenic modifications. We therefore interrogated our RNA-seq dataset for potential transcriptional modifications in genes associated with adipogenesis and found transcript level changes in CEBPα, LEPR, ID4 and STEAP4 (Figure 4F–I). Surprisingly, each of these genes involved in promoting adipogenesis had lower expression in MSCs from people with OP versus those from AMC. To further investigate, we progressed to assess the adipogenic potential of MSCs isolated from donors living with and without OP. MSCs were induced to differentiate with adipogenic medium, and their lipid content observed using Oil Red O staining. Overall, the lipid content per cell appeared to be comparable in both cultures but with fewer cells entering the adipogenic lineage in cultures from donors with OP in comparison to the homogenous distribution of lipid-filled MSC-derived adipocytes in induced cultures from AMC donors (Figure 4J). Quantification of Oil Red O was by absorbance measurements of extracted stain, and it demonstrated that MSCs isolated from donors living with OP had less Oil Red O retention than MSCs isolated from AMC donors (Figure 4K). Considering the high variability in the range of adipogenic capacity in both cohorts, a relationship to donor age was assessed. Increased age was associated with a reduced adipogenic potential in MSCs from donors with OP only (Figure 4L and M). These findings indicate that MSC adipogenesis is reduced in people with OP in an age-dependent manner and that those changes are stable through ex vivo expansion.
Discussion
OP can be a silent disease, not diagnosed until the presentation of a bone fracture. The global number of annual OP-associated fractures was last estimated in 2006 at 9 million [23], this number will now be considerably higher considering the increasing rates of OP globally, with recent incidence rates in Ireland reported at 1,000 per 100,000 [24]. We estimate there were > 19 million fracture incidents in women over 65 years of age in 2019, which equates to 11% of total fractures across demographics (calculated using data from [25, 26]). More than 90% of fractures in this age/gender category are linked to low BMD [27], and additional OP-associated fractures occur outside this category in men (risk of fracture triples from age 70 to 90) [26] and in younger women [28]. These fragility fractures are associated with high rates of morbidity and mortality, with hazard ratios for mortality as high as 10.98 [29]. The prevalence and severity of these OP-associated adverse outcomes demonstrates the need for further research and improved therapeutics.
This study investigated the potential role for MSC dysfunction in OP by assessing transcriptional and functional modifications in bone marrow MSCs from people with and without OP. We found that people with OP had transcriptionally modified MSCs that were fewer in number, and had reduced osteogenic and adipogenic differentiation potential, with no observed loss of capacity for proliferation. Altered transcript levels in osteogenic and adipogenic pathways had functional consequences on the ability of MSCs from people with OP to differentiate in the osteoblastic and adipogenic lineages. Early studies in this area indicated reduced osteogenic potential and increased adipogenic differentiation of MSCs from people with OP [12, 13, 30]. While an imbalance between adipogenesis and osteogenesis is often cited as a mechanistic explanation of OP [31, 32] BMI, bone marrow adiposity and BMD are overall positively correlated [6–8, 33]. Decreased bone quality occurs at both extremes of body weight with frailty at the lower end and low-quality dense bone at excess body weights [19, 33]. Therefore, the parallel reduction in both the osteogenic and adipogenic capacity of MSCs from people with OP observed in this study aligns with the correlative nature of bone and adipose in overall metabolic physiology. This is an important finding as it indicates that efforts to switch MSC differentiation preference from the adipose lineage to the osteogenic lineage may not be useful in developing therapeutics for OP.
That we recorded fewer MSCs in the bone marrow of people with OP compliments previous studies which described inhibited short term expansion capacity in bone marrow derived human MSCs, and decreased peripheral blood MSC number in people with OP [12, 30]. We did not observe any ex vivo alteration to proliferative capacity of human MSCs from people with OP. However, proliferation capacity as a variable is readily altered by culture conditions. The conditions in the present study were optimised for regenerative medicine, and therefore for MSC expansion in the context of their therapeutic application. The potential idea that an in vivo retardation of MSC proliferative capacity can be rescued ex vivo, is an attractive model for autologous MSC treatment in OP.
Current therapeutic strategies to treat OP primarily utilise pharmaceuticals such as bisphosphonates and denosumab that inhibit osteoclastic resorption [3, 34]. Prescription and adherence rates for these medications are low, and therapeutics with increased tolerability are required [3, 34]. MSCs harbour great promise as a cellular therapeutic and could be of significant value in OP due to their ability to regulate both osteoblasts and osteoclasts, and their potential for longer-acting benefit with fewer side-effects than current treatments [35]. To date, one clinical trial has taken place using autologous bone marrow derived human MSCs as a treatment for OP. No adverse effects were reported and there was an indication that the therapy led to reduced pain in the treated patients, with no new fractures reported during the study period [36].
This study identifies new targets with potential application in the development of OP treatment. Novel targets with known links to osteogenesis identified in this study as differentially expressed in OP include P2RY6, AJAP1, PRKG1, ARHGAP32, EPHB6, HAUS5, WAVE/WASF1 and Reelin. P2YR6, which has been shown to promote bone resorption, was increased here in people with OP [37], while we found PTDGS, which contributes to inhibition of osteoclastogenesis, is lower in people with OP compared to AMC [38]. These data support the role of MSCs in modifying osteoclast behaviour. Other identified targets are supported by previous studies of BMD—SMOC1, NPR3 (by GWAS) and TEP1 (by RNA-seq) [39–41]. SMOC1 in particular is a promising target as it acts downstream of RUNX2, a known master regulator of osteogenesis. While therapeutic upregulation of RUNX2 raises concerns regarding potential adverse effects including increased cancer and osteoarthritis risk [42, 43], SMOC1 expression is instead associated with more favourable cancer outcomes [44, 45]. Similarly to RUNX2, oncogenic properties are also described for other previously identified genes, e.g., EFNB2 [46]. Therefore, the identification of novel OP-associated genes in this study, especially those with described associations to BMD (e.g., SMOC1, NPR3, TEP1), has important implications for future safe and effective OP therapeutic development. Considering the observed functional changes, along with the transcriptional changes suggesting dysfunction in the way MSCs from people with OP regulate processes by exerting control on surrounding cell populations, our data indicate allogenic or ex vivo modified autologous MSCs may hold greatest promise for the development of MSCs as a therapeutic for OP.
It is worth highlighting that donor ages in the RNA-seq evaluation and proliferation assay are statistically significantly different from one another (Table S1), even though all donors were mature in age. The findings of the RNA-seq study links directly with the osteogenic and adipogenic differentiation assays. Therefore, the changes identified in the transcriptome persist in functional differences between study cohorts, regardless of donor age. Similarly, there was no change in proliferative activity between donor cohorts, indicating that the age of donor was irrelevant. It is therefore concluded that the value of the current study’s findings remain strong. Herein data is presented describing a mature population of human donors, enabling a direct link between these in vitro assays and future clinical application.
Therapeutic development will require future interventional and larger scale observational research studies due to inherent limitations of this study. The current study includes minimal information on all donors, in line with GDPR legislation and the study’s ethical approval. Future studies would benefit from acquiring a broader medical history including medication use and BMI of donors. Further, conditions common in the age category of the donors [66–80 years old, e.g., type 2 diabetes, cardiovascular disease, osteoarthritis, dementia and chronic obstructive pulmonary disease (COPD)] were not categorised as exclusion criteria in this study due to their prevalence. The prevalence is such that excluding donors with those morbidities had the potential to render our results less relevant to the population for whom therapeutic development is aimed, as well as greatly reducing the samples available for study. A larger study with more detailed donor medical history would be of benefit to assess the impact of potentially confounding variables. Interventional analyses targeting the identified genes will further strengthen their potential as targets for therapy. Importantly, overexpression of SMOC1 in human bone marrow MSCs has been shown to increase both proliferation and osteoblast differentiation [47, 48]. Transplantation of such modified MSCs in a mouse model of OP would be an important step in translating these findings toward clinic. As an imprinted gene, SMOC1 has high dosage sensitivity with the potential to act as a mediator between environmental inputs and osteogenic turnover rate [49]. For example, SMOC1 methylation is increased by triclosan, an antibacterial chemical with higher burden in women that correlates with reduced BMD [50, 51]. Rectifying such aberrant loss of osteogenic gene expression may prove an effective method for restoring BMD in people with OP.
Overall, these data support an important role for MSCs in the development of OP. Understanding the role of MSC dysfunction in OP development provides foundational knowledge for future therapies. Because ex vivo modification of MSCs could increase their potential as therapeutics identifying targets for modification is crucial in their successful translation to clinic. This study expands our understanding of the aetiology of OP, as well as providing a broader knowledge base to allow the development of future therapies for OP, either targeting the maintenance of the human MSC population in situ or utilisng them as an ex vivo expanded therapy.
Abbreviations
AJAP1: | adherens junctions associated protein 1 |
AMC: | age-matched control |
ARHGAP32: | Rho GTPase activating protein 32 |
BMD: | bone mineral density |
BMI: | body mass index |
CEBPα: | CCAAT enhancer binding protein alpha |
CFU-F: | colony forming unit-fibroblast |
CFU-O: | colony forming unit-osteoblast |
EFNB2: | ephrin B2 |
EPHB6: | EPH receptor B6 |
FAM193B: | family with sequence similarity 193 member B |
FBS: | fetal bovine serum |
GO: | Gene Ontology |
HAUS5: | HAUS augmin like complex subunit 5 |
ID4: | inhibitor of DNA binding 4 |
LEPR: | leptin receptor |
MASP1: | MBL associated serine protease 1 |
MNCs: | mononuclear cells |
MSCs: | mesenchymal stromal cells |
NEFL: | neurofilament light chain |
NHS: | actin remodeling regulator |
NPR3: | natriuretic peptide receptor 3 |
OP: | osteoporosis |
P2RY6: | pyrimidinergic receptor P2Y6 |
PRKG1: | protein kinase CGMP-dependent 1 |
PS: | penicillin-streptomycin |
PTDGS: | prostaglandin D2 receptor |
RNA-seq: | ribonucleic acid sequencing |
SMOC1: | SPARC related modular calcium binding 1 |
STEAP4: | STEAP4 metalloreductase |
TEP1: | telomerase associated protein 1 |
TRIT1: | TRNA isopentenyltransferase 1 |
UBXN8: | UBX domain protein 8 |
ZNF555: | Zinc finger protein 555 |
Supplementary materials
The supplementary material for this article is available at: https://www.explorationpub.com/uploads/Article/file/100746_sup_1.pdf.
Declarations
Acknowledgments
The authors would like to thank the bone marrow donors for their generosity; L. Howard and J. McMahon for insightful scientific discussions; C. McHale for transporting bone marrow samples; A. Keane, C. Lyons and C. Murray for their work in vitro; J. McCabe, A. Devitt, F. Shannon, and O. Godkin for their support of and active contribution to the donation programme; and A. Simpkin for his statistical expertise. Technical and consultative support for flow cytometry experiments was provided by Dr Shirley Hanley of the NUI Galway Flow Cytometry Core Facility. The authors also acknowledge the facilities and scientific and technical assistance of the Anatomy Microscopy and Imaging core facility at the University of Galway.
Author contributions
FCC: Methodology, Validation, Formal analysis, Investigation, Data curation, Writing—original draft, Writing—review & editing, Supervision, Project administration. CS: Methodology, Validation, Investigation, Data curation, Writing—original draft, Writing—review & editing. KT: Investigation. ASA: Methodology, Formal analysis, Investigation. CGM: Methodology, Resources, Writing—review & editing, Project administration. SRK and WC: Resources, Writing—review & editing. KGW: Methodology. TO: Conceptualization, Formal analysis, Resources, Writing—original draft, Writing—review & editing, Supervision, Project administration, Funding acquisition. CMC: Conceptualization, Methodology, Validation, Formal analysis, Investigation, Resources, Data curation, Writing—original draft, Writing—review & editing, Supervision, Project administration, Funding acquisition. All authors have read and agreed to the published version of the manuscript.
Conflicts of interest
Timothy O’Brien is a founder, director, and equity holder in Orbsen Therapeutics. The other authors declare that they have no conflicts of interest.
Ethical approval
All procedures complied with the Declaration of Helsinki and were approved by the Clinical Research Ethics Committee of Galway University Hospitals (C.A. 1621 from 3 November 2016).
Consent to participate
Informed consent to participate in this study was obtained from all participants.
Consent to publication
Not applicable.
Availability of data and materials
The sequencing data described in this manuscript have been deposited in NCBI’s Gene Expression Omnibus and are accessible through GEO Series accession number GSE189524 (https://www.ncbi.nlm.nih.gov/geo/query/acc.cgi?acc=GSE189524). So long as it complies with our ethical approval, other raw data supporting the conclusions of this manuscript will be made available by the authors, without undue reservations, to any qualified researcher. Requests for data should be made to cynthia.coleman@universityofgalway.ie.
Funding
This research was funded by the Health Research Board and Diabetes Ireland Research Alliance under the MRCG-HRB Joint Funding Scheme, grant number [HRB-MRCG-2016-2]; all flow cytometry experiments were performed in the NUI Galway Flow Cytometry Core Facility which is supported by funds from NUI Galway, Science Foundation Ireland, the Irish Government’s Programme for Research in Third Level Institutions, Cycle 5 and the European Regional Development Fund. The funders had no role in study design, data collection and analysis, decision to publish, or preparation of the manuscript.
Copyright
© The Author(s) 2024.