Abstract
Glioma is a highly aggressive brain cancer associated with significant mortality. Despite advances in diagnostic and therapeutic strategies, the prognosis for glioma patients remains poor due to limited diagnostic accuracy and monitoring capabilities. Translocator protein (TSPO) is a mitochondrial protein implicated in various cancers, including glioma, where it plays a significant role in cell survival, proliferation, and chemo-resistance. This review article aimed to comprehensively analyze the role of TSPO in glioma, particularly its potential applications in enhancing diagnostic methods and therapeutic strategies. Molecular imaging techniques have emerged as promising tools for non-invasive diagnosis, disease progression monitoring, and treatment selection of gliomas. A comprehensive literature review was conducted to explore TSPO’s expression patterns, biological functions, and applications in molecular imaging. Studies utilizing positron emission tomography (PET), single photon emission computed tomography (SPECT), magnetic resonance imaging (MRI), and other imaging modalities were included. TSPO is overexpressed in glioma cells, particularly in high-grade tumors, correlating with tumor aggressiveness and patient prognosis. TSPO-targeted imaging agents demonstrate high specificity and sensitivity for glioma detection, positioning TSPO as a promising marker for accurate diagnosis and therapeutic monitoring. Future studies should focus on optimizing TSPO imaging protocols, validating their clinical utility, and exploring combined imaging modalities to improve diagnostic precision.
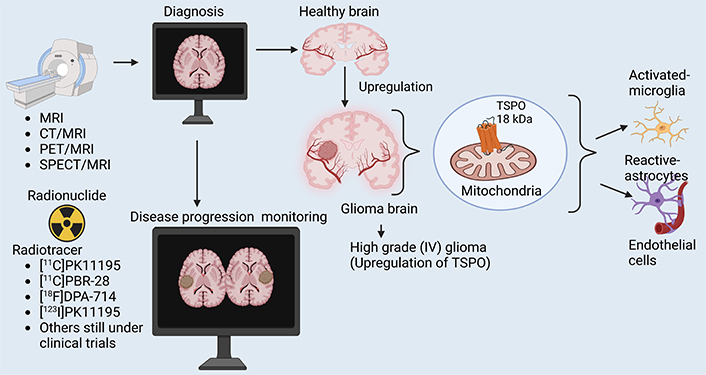
Keywords
Translocator protein, glioma, molecular imaging, diagnosis, disease progression monitoring, targeted therapyIntroduction
Definition and pathogenesis of glioma
Gliomas are brain tumors arising from central nervous system (CNS) glial cells, classified by the World Health Organization (WHO) based on histological and molecular traits into astrocytomas, oligodendrogliomas, and ependymomas [1–4]. Gliomas are the most common type of primary brain tumor, accounting for approximately 75% of all brain tumors [4, 5].
The pathogenesis of gliomas involves a multistep process characterized by genetic mutations, epigenetic alterations, and interactions within the tumor microenvironment. Key genetic abnormalities often include mutations in the IDH1/IDH2 genes, which are critical for cellular metabolism and contribute to the accumulation of oncometabolite 2-hydroxyglutarate (2-HG), promoting tumorigenesis and altering cellular differentiation [6]. Additionally, loss of the tumor suppressor gene p53 and alterations in the retinoblastoma (Rb) signaling pathway are commonly observed in higher-grade gliomas, leading to uncontrolled cellular proliferation and survival [7, 8]. Epigenetic modifications, such as aberrant DNA methylation patterns, further drive glioma progression and contribute to the heterogeneity of these tumors [8]. Moreover, the tumor microenvironment, including the presence of immune cells and extracellular matrix components, plays a significant role in glioma development and therapy resistance [9]. Understanding these intricate mechanisms is crucial for developing targeted therapies and improving treatment outcomes for glioma patients.
Diagnosis and disease progression monitoring of glioma with traditional methods
Diagnosing and monitoring glioma remains challenging, especially using traditional imaging methods (Table 1). Traditional methods of diagnosing and monitoring glioma progression in patients include: (1) MRI (magnetic resonance imaging): MRI is the standard imaging modality for diagnosing and monitoring glioma progression; however, it has several limitations in distinguishing true tumor progression from treatment-related effects [10]. A major challenge is pseudoprogression, where post-radiotherapy inflammatory changes mimic tumor growth, particularly in patients treated with temozolomide and radiotherapy [11]. Conversely, pseudoresponse occurs in patients receiving anti-angiogenic therapies (e.g., bevacizumab), where reduced contrast enhancement gives a false impression of tumor shrinkage despite ongoing malignancy [12]. Another limitation is the inability of conventional MRI to accurately delineate infiltrative tumor margins, as glioma cells extend beyond the contrast-enhancing region, leading to underestimation of residual disease [13]. Furthermore, MRI has poor specificity in differentiating tumor recurrence from radiation necrosis, as both conditions exhibit similar imaging characteristics [14]. Low-grade gliomas and non-enhancing tumor components are also difficult to monitor using standard MRI, necessitating advanced imaging techniques [15]. While diffusion-weighted imaging (DWI), dynamic susceptibility contrast (DSC) perfusion MRI, and magnetic resonance spectroscopy (MRS) improve diagnostic accuracy, they still lack the molecular-level precision required for accurate assessment [16]. Translocator protein (TSPO) positron emission tomography (PET) imaging has emerged as a promising complementary tool for distinguishing active tumor growth from inflammatory changes, but its integration into routine clinical practice is still evolving [17]. (2) Computed tomography (CT) scans: CT scans use X-rays and computer technology to produce detailed brain images. While CT scans are widely used, they have limitations, such as (i) radiation exposure: CT scans use ionizing radiation, which can increase the risk of cancer in patients with a history of radiation exposure; (ii) limited sensitivity: CT scans may not detect small tumors or early signs of recurrence; (iii) limited spatial resolution: CT scans resolution may not be sufficient to detect small changes in tumor size or shape. (3) A neurological examination is a non-invasive method that assesses the patient’s symptoms, cognitive function, and motor skills, providing valuable information on tumor progression [18]. (4) Stereotactic biopsy is an invasive method that involves collecting tissue samples from the brain to identify genetic markers and determine tumor grade. Still, it is limited by its invasiveness and potential risks [19]. (5) Contrast-enhanced imaging, such as MRI or CT scans, uses contrast agents to highlight tumor activity and detect changes in tumor size and shape, allowing for monitoring of tumor progression and response to treatment [20]. While these methods are widely used, they have limitations, including false-negative results and incomplete sampling of the tumor. For example, a neurological examination may not detect subtle changes in cognitive function or motor skills, while a stereotactic biopsy may not capture the entire extent of the tumor [21, 22]. Newer imaging modalities, such as functional MRI and MRS, may offer improved diagnostic accuracy and reduced radiation exposure. Further research is needed to develop more accurate and non-invasive methods for monitoring glioma patients. The traditional methods for diagnosis and disease progression monitoring, as well as their merits and demerits, are presented in Table 1.
Traditional methods for diagnosis and disease progression monitoring with merits and demerits
Method | Description | Merits | Demerits | Reference(s) |
---|---|---|---|---|
MRI | Uses strong magnets and radio waves to create detailed images of the brain and tumor. | Non-invasive, high-resolution imaging can distinguish between tumor types. | High cost, requires careful interpretation, and may not show immediate changes. | [23, 24] |
CT | CT scan combines X-ray images taken from different angles to create cross-sectional brain images. | It is widely available, quicker than MRI, and effective for detecting larger tumors or blood. | A lower resolution than MRI involves radiation exposure and is less effective for soft tissues. | [23, 25] |
Neurological examination | Clinical evaluation by a healthcare professional to assess cognitive function, reflexes, motor skills, and sensory abilities. | Immediate feedback on neurological function, no imaging required. | Subjective, may not detect small changes in tumor status. | [26] |
Biopsy (stereotactic) | A minimally invasive procedure to obtain tissue samples from the tumor for histopathological analysis. | Provides a definitive diagnosis and information about the tumor type. | Invasive procedures with associated risks may not represent the entire tumor heterogeneity. | [27, 28] |
Contrast-enhanced imaging | Use of contrast agents in MRI or CT scans to enhance the visibility of tumor boundaries and blood supply. | It improves differentiation between tumor and normal tissue and highlights tumor vascularity. | Contrast agents can cause allergic reactions or kidney issues in some patients. | [29] |
CT: computed tomography; MRI: magnetic resonance imaging
Molecular imaging techniques for glioma
Molecular imaging techniques offer a promising approach for diagnosis and monitoring disease progression in glioma patients. These techniques utilize targeted probes that bind specifically to biomarkers that are overexpressed in glioma [30]. One such molecular imaging technique is PET imaging. PET imaging uses radiotracers that bind to specific molecular targets. For example, the radiotracer [18F]FLT ([18F]3'-deoxy-3'-fluorothymidine) has been shown to accumulate in glioma cells and can be used to monitor tumor proliferation and growth [31]. As stated by Schiepers et al. [32], in a study published in the Journal of Nuclear Medicine, [18F]FLT PET was used to monitor disease progression in patients with glioblastoma, the most aggressive form of glioma. The study showed that [18F]FLT PET imaging could detect changes in tumor biology early, allowing for more effective treatment and monitoring [31, 33]. Much as PET scans use small amounts of radioactive material to produce images of metabolic activity in the brain [31], PET scans are sensitive for detecting metabolic changes. However, they have limitations, such as (1) radiation exposure: PET scans use ionizing radiation, which can increase the risk of cancer in patients with a history of radiation exposure; (2) limited spatial resolution: PET scan resolution may not be sufficient to detect small changes in tumor size or shape; (3) high cost: PET scans are expensive and may not be available in all hospitals [34].
TSPO PET imaging provides a unique advantage over traditional imaging modalities like MRI and CT by allowing the visualization of early molecular changes in glioma biology associated with tumor proliferation and inflammatory processes. Unlike MRI and CT, which primarily offer anatomical information, TSPO PET detects the upregulation of TSPO expression, often linked to activated microglia and macrophages within the tumor microenvironment, which occurs even prior to significant tumor growth [35]. This early detection capability is crucial for understanding the underlying biological behavior of gliomas, as it may provide insights into tumor aggressiveness and response to therapies [36]. As stated by Simmons et al. [37], TSPO PET imaging can serve as a biomarker for monitoring therapeutic efficacy, thereby enhancing the precision of glioma management [35]. Given these advantages, TSPO PET emerges as a promising tool for early glioma diagnosis and disease progression monitoring, complementing traditional imaging approaches.
Besides TSPO PET, several other molecular PET imaging techniques have been developed to monitor the progression of glioma. One widely used approach is amino acid PET imaging, particularly with radiotracers such as [18F]FET (O-(2-[18F]fluoroethyl)-L-tyrosine) and [11C]MET (L-[methyl-¹¹C]methionine), which take advantage of the increased amino acid transport in gliomas to provide better tumor delineation and grading [38–40]. Additionally, as stated by Verger et al. [38], [18F]FDOPA (6-[18F]fluoro-L-3,4-dihydroxyphenylalanine) has been utilized to assess glioma progression and response to therapy by targeting the upregulated L-type amino acid transporter 1 (LAT1) in tumor cells. Another significant PET imaging strategy involves glucose metabolism with [18F]FDG (2-[¹⁸F]fluoro-2-deoxy-D-glucose) [38, 41]. However, its clinical utility in gliomas is limited due to high background uptake in normal brain tissue [42]. Beyond metabolism-based tracers, receptor-targeted PET imaging has also gained interest, with [18F]-PSMA (prostate-specific membrane antigen)-1007 and [68Ga]PSMA PET showing promise in identifying glioblastoma due to PSMA expression in neovascular endothelium [43]. Furthermore, hypoxia-targeted PET imaging, using [18F]FMISO ([¹⁸F]fluoromisonidazole), helps in assessing tumor hypoxia, a key factor in glioma aggressiveness and resistance to therapy [41]. These diverse molecular PET techniques complement TSPO PET by offering insights into glioma biology, including metabolism, amino acid transport, receptor expression, and tumor hypoxia, ultimately enhancing tumor characterization and treatment monitoring.
Another molecular imaging technique is single photon emission CT (SPECT) imaging, which uses radiolabeled molecules that emit gamma radiation, which can be detected by SPECT imaging. SPECT is an important functional imaging technique used in the diagnosis and monitoring of glioma progression, particularly when combined with radiotracers that target tumor metabolism and proliferation. SPECT imaging offers advantages in detecting tumor viability, distinguishing recurrent glioma from post-treatment effects, and assessing response to therapy [17, 44]. Commonly used radiotracers include 99mTc-MIBI (technetium-99m methoxyisobutylisonitrile), which accumulates in viable tumor cells due to increased mitochondrial activity, and 201Tl (thallium-201)-chloride, which reflects cellular proliferation and membrane integrity [17, 45, 46]. Additionally, SPECT with amino acid tracers such as 123I-α-methyl-L-tyrosine (123I-IMT) provides enhanced specificity by targeting glioma-specific metabolic pathways [47, 48]. Compared to MRI, SPECT can differentiate between tumor recurrence and radiation necrosis, reducing diagnostic uncertainty [17, 49]. However, its lower spatial resolution compared to PET and MRI limits its standalone use, making it most effective when integrated into multimodal imaging approaches [50]. Despite these limitations, advances in hybrid SPECT/CT imaging and novel radiotracers are improving their clinical utility in glioma management [50].
Molecular imaging techniques have the potential to detect early changes in tumor biology and provide valuable information for treatment planning and monitoring. Further research is needed to understand the potential of these techniques fully and to develop new molecular imaging agents for the detection and monitoring of gliomas. Early detection capability in gliomas is crucial for understanding the underlying biological behavior of gliomas, as it may provide insights into tumor aggressiveness and response to therapies [51].
TSPO
TSPO, a conserved protein in the outer mitochondrial membrane, is also known as the peripheral benzodiazepine receptor (PBR) or the mitochondrial benzodiazepine receptor (MBR) [51, 52], which modulates mitochondrial function, cholesterol transport, and inflammatory responses [53]. TSPO has a variety of biological functions, such as steroid hormone synthesis, apoptosis, cell proliferation, porphyrin and heme transport, anion transport, and immunomodulation [36, 51–56]. Additionally, TSPO is upregulated in various cancers and neurodegenerative diseases, i.e., breast, prostate, colon, brain tumors, Alzheimer’s disease, and Parkinson’s disease. TSPO expression is linked to tumor progression and poor survival rates in cancer patients [36, 51, 57]. In glioma pathogenesis, TSPO has been shown to be involved in promoting tumor growth and progression [51].
Studies have suggested that TSPO expression is increased in glioma cells and that it may contribute to the resistance of glioma cells to chemotherapy and radiotherapy. Additionally, TSPO has been implicated in the pathogenesis of gliomas, although the exact role of TSPO in gliomagenesis is still not fully understood [51]. Some of the key points discussing the involvement of TSPO in gliomas include: (1) expression in glioma cells, as proposed by Ammer et al. [51], TSPO is expressed in glioma cells, with varying levels of expression reported across different glioma subtypes and grades. Higher TSPO expression has been observed in high-grade gliomas (III–IV), such as glioblastoma, compared to low-grade gliomas (I–II), such as diffuse astrocytoma, oligodendroglioma, etc. [58]. (2) Involvement in glioma cell proliferation and survival: TSPO has been suggested to play a role in promoting glioma cell proliferation and survival. Activation of TSPO signaling has been associated with increased cell growth, resistance to apoptosis, and enhanced migration and invasion of glioma cells [51]. (3) Relationship with mitochondrial function, where TSPO is primarily localized to the mitochondria and is involved in regulating mitochondrial functions, such as bioenergetics, metabolism, and reactive oxygen species (ROS) production. Dysregulation of mitochondrial function is a hallmark of gliomas, and TSPO may contribute to mitochondrial dysfunction in glioma cells [59]. (4) Interaction with signaling pathways in which TSPO has been reported to interact with various signaling pathways implicated in glioma pathogenesis, such as the PI3K/AKT/mTOR (phosphoinositide 3-kinase/protein kinase B/mammalian target of rapamycin) pathway, MAPK/ERK (mitogen-activated protein kinase/extracellular signal-regulated kinase) pathway, and NF-κB (nuclear factor kappa-light-chain-enhancer of activated B cells) pathway. Activation of these pathways can lead to increased glioma cell proliferation, survival, migration, and resistance to therapy [60, 61] (Figure 1). (5) Potential as a therapeutic target: given its expression in glioma cells and its role in promoting glioma progression, TSPO has been suggested as a potential therapeutic target for glioma treatment. Inhibition of TSPO activity or expression may offer a promising strategy to impair glioma cell growth and enhance the efficacy of current treatment modalities [51]. (6) Imaging biomarker: TSPO expression in glioma tumors can also serve as a potential imaging biomarker for the non-invasive detection and monitoring of glioma progression [35, 62]. Imaging techniques using radiolabeled ligands that bind to TSPO have been developed for visualizing neuroinflammation and monitoring the progression of gliomas. PET using a radiolabeled TSPO probe has allowed non-invasive and reliable investigation of the role of TSPO in neuropathological damage and tumor progression [57]. TSPO-influenced signaling pathways in glioma cells leading to tumor progression are illustrated in Figure 1.
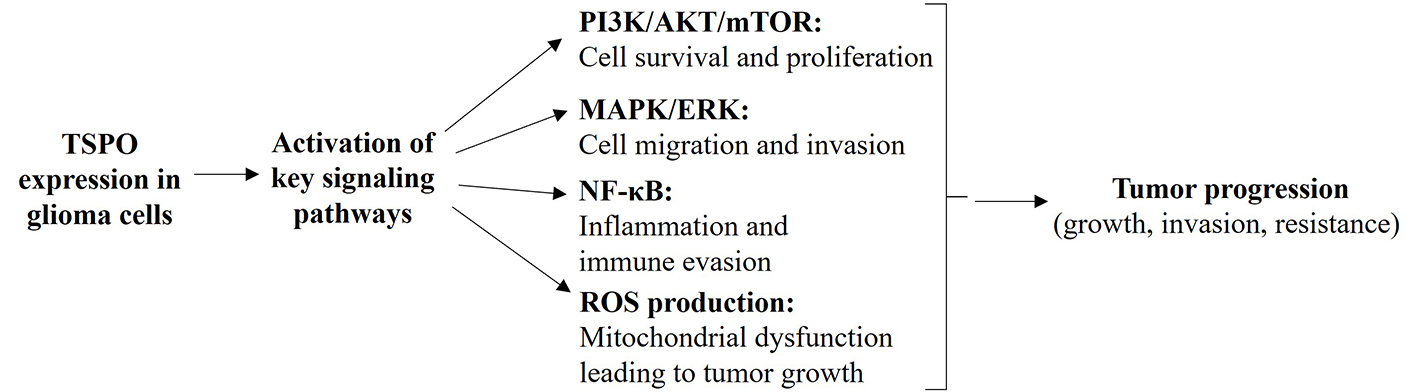
TSPO-influenced signaling pathways in glioma. TSPO is overexpressed in glioma cells, leading to activation of key signaling pathways: (1) PI3K/AKT/mTOR pathway: effect: promotes cell survival and proliferation; outcome: leads to increased tumor growth. (2) MAPK/ERK pathway: effect: enhances cell migration and invasion; outcome: facilitates tumor spread. (3) NF-κB pathway: effect: activates inflammatory responses and contributes to immune evasion; outcome: supports tumor progression and resistance to immune attacks. (4) ROS production: effect: causes mitochondrial dysfunction; outcome: promotes tumor growth and resistance to apoptosis. The overall impact on glioma cells: enhanced cell survival and proliferation, increased migration and invasion, inflammation and immune evasion, and resistance to therapy. MAPK/ERK: mitogen-activated protein kinase/extracellular signal-regulated kinase; NF-κB: nuclear factor kappa-light-chain-enhancer of activated B cells; PI3K/AKT/mTOR: phosphoinositide 3-kinase/protein kinase B/mammalian target of rapamycin; ROS: reactive oxygen species; TSPO: translocator protein
Role of TSPO in glioma development
The role of TSPO in glioma development (Figure 2) highlights the expression levels of TSPO in glioma, the effects of TSPO on tumor proliferation and migration, and the regulation of TSPO expression in glioma, all of which contribute to glioma development.
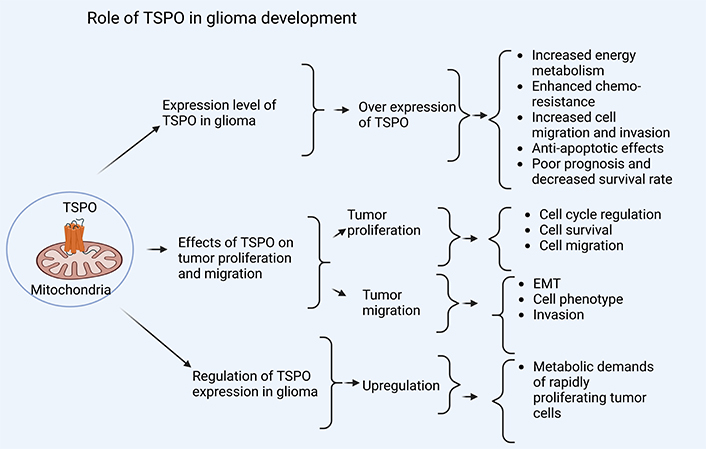
Role of TSPO in glioma development. The role of TSPO in glioma development is linked to the expression level of TSPO in glioma, as overexpression is associated with increased energy metabolism, enhanced chemo-resistance, increased cell migration and invasion, anti-apoptotic effects, poor prognosis, and decreased survival rate. Similarly, the effects of TSPO on tumor proliferation and migration, whereby tumor proliferation is associated with cell cycle regulation, cell survival, and cell migration, and tumor migration is associated with EMT, cell phenotype, and invasion. Furthermore, regulation of TSPO expression in glioma, for instance, upregulation of TSPO, is associated with metabolic demands of rapidly proliferating tumor cells. EMT: epithelial-mesenchymal transition; TSPO: translocator protein. Created in BioRender. Mulumba, J. (2025) https://BioRender.com/s89r048
Expression level of TSPO in glioma
TSPO plays an important role in various physiological processes, including energy metabolism, neurotransmission, cell proliferation, apoptosis, inflammation, and cell survival [53]. In glioma, TSPO has been implicated in tumor progression, invasion, and resistance to chemotherapy [63]. Studies have shown that TSPO is overexpressed in glioma cells compared to normal brain tissue. A study published in the Journal of Neuro-Oncology found that TSPO expression was significantly higher in glioblastoma multiforme (GBM) tissue than in normal brain tissue [51]. Another study published in the Journal of Neurochemistry found that TSPO expression was increased in glioma cells compared to normal astrocytes [59, 64].
Overexpression of TSPO in glioma cells has been linked to several mechanisms that contribute to tumor progression, including: (1) increased energy metabolism: TSPO is involved in the transport of reducing equivalents across the mitochondrial inner membrane, which can lead to increased energy production and enhanced cell growth; (2) enhanced chemo-resistance: TSPO has been shown to play a role in the transport of chemotherapeutic drugs out of the cell, which can contribute to the development of resistance to chemotherapy; (3) increased cell migration and invasion: TSPO has been implicated in the regulation of cell migration and invasion, which are key processes involved in glioma progression; (4) anti-apoptotic effects: TSPO has been shown to inhibit apoptosis in glioma cells, which can contribute to their survival and proliferation [62, 65]. The TSPO-targeted therapies for glioma (Figure 3) highlight different types of therapeutic interventions in the treatment of glioma, both current and future.
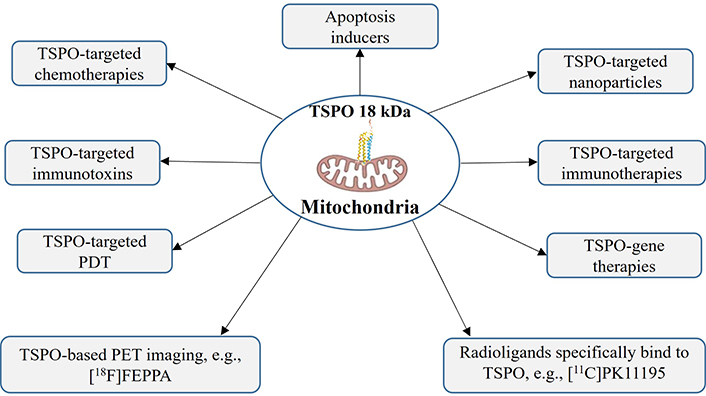
TSPO-targeted therapies for glioma. Illustrates various therapeutic strategies targeting TSPO in glioma treatment, including apoptosis inducers, TSPO-targeted chemotherapies, TSPO-targeted immunotoxins, TSPO-targeted PDT, TSPO-based PET imaging (e.g., [18F]FEPPA), TSPO-targeted nanoparticles, TSPO-targeted immunotherapies, TSPO-gene therapies, and radioligands specifically bind to TSPO (e.g., [11C]PK11195). [11C]PK11195: [11C]N-butan-2-yl-1-(2-chlorophenyl)-N-methylisoquinoline-3-carboxamide; [18F]FEPPA: [¹⁸F]N-(2-(2-fluoroethoxy)benzyl)-N-(4-phenoxypyridin-3-yl)acetamide; PDT: photodynamic therapy; PET: positron emission tomography; TSPO: translocator protein
Regarding the clinical significance of TSPO expression in glioma, several studies have demonstrated that high levels of TSPO expression are associated with poor prognosis and reduced survival rates. A study published in the Journal of Neuro-Oncology found that patients with GBM who had high levels of TSPO expression had a significantly shorter median survival time than those with low TSPO expression levels [58, 66].
Targeting TSPO has been proposed as a potential therapeutic strategy for glioma treatment. For example, some studies have shown that inhibitors of TSPO can enhance the effectiveness of chemotherapy and radiation therapy by increasing drug uptake and reducing resistance mechanisms. Additionally, some studies have explored the use of TSPO as a biomarker for diagnosing and monitoring glioma patients [67]. This suggests that combining TSPO-targeting therapy with chemotherapy may be an effective treatment strategy. Studies indicated that TSPO expression tends to increase with the advancing grade of gliomas (low to high). The characteristic features of the effect of TSPO expression on glioma progression.
The effect of TSPO expression on glioma grade and progression
The expression levels of TSPO in glioma have been shown in Table 2, highlighting the characteristics and different grades of glioma cells.
Effect of TSPO expression on glioma progression
Characteristic | Low TSPO expression(grades I–II) | High TSPO expression(grades III–IV) | Reference |
---|---|---|---|
Cell proliferation | Low proliferation rates, slower tumor growth. | High proliferation rates and rapid tumor growth. | [51] |
Apoptosis | Increased apoptosis, less aggressive behavior. | Reduced apoptosis and enhanced tumor survival. | [68] |
Invasion potential | Low invasiveness and well-defined tumor margins. | Higher invasive capabilities and infiltrative tumor spread. | [69] |
Inflammation response | Mild inflammatory response, less immune evasion. | Strong pro-inflammatory environment, immune system suppression. | [70] |
Chemo-resistance | More susceptible to therapy. | Increased drug resistance and poor prognosis. | [68] |
TSPO: translocator protein
Gliomas are classified into low-grade (I–II) and high-grade (III–IV) tumors based on their malignancy and progression. Low-grade gliomas (I–II): slow-growing, less invasive, and often have lower TSPO expression. These include pilocytic astrocytoma (grade I) and diffuse astrocytoma (grade II). High-grade gliomas (III–IV): aggressive, highly invasive, and characterized by high TSPO expression (Table 2). These include anaplastic astrocytoma (grade III) and GBM (grade IV), the most aggressive form [71]. Furthermore, the elevated expression of TSPO has been explored as a potential biomarker for glioma prognosis, with studies suggesting that higher TSPO levels might correlate with shorter patient survival times [51].
Effects of TSPO on tumor proliferation and migration
Some of the effects of TSPO on tumor proliferation and migration are (1) effects on tumor proliferation: (i) cell cycle regulation, where TSPO has been shown to regulate cell cycle progression by modulating the activity of cyclin-dependent kinases (CDKs) and their inhibitors. The overexpression of TSPO in glioma cells can promote cell proliferation by activating CDKs and reducing the activity of CDK inhibitors [62]. (ii) Cell survival, where TSPO can promote cell survival by inhibiting apoptosis through the activation of anti-apoptotic signaling pathways, such as the PI3K/AKT pathway [62]. (iii) Cell migration, whereby TSPO can also promote cell migration by regulating the activity of cytoskeletal proteins, such as actin and microtubules, which are essential for cell migration [72]. (2) Effects on tumor migration: (i) epithelial-mesenchymal transition (EMT), where TSPO has been shown to promote EMT, a process by which epithelial cells acquire a mesenchymal phenotype, which is associated with increased migratory and invasive capabilities. (ii) Cell adhesion, where TSPO can regulate cell adhesion by modulating the activity of adhesion molecules, such as integrins and cadherins, which are essential for cell-cell and cell-matrix interactions. (iii) Invasion, in which TSPO can promote tumor invasion by regulating the activity of matrix metalloproteinases (MMPs), which are responsible for degrading the extracellular matrix and promoting tumor invasion [73].
Mechanisms underlying the effects of TSPO on glioma development include: (1) hypoxia: TSPO is upregulated in response to hypoxia, which is a common feature of glioma. Hypoxia-induced TSPO expression promotes cell survival and proliferation, contributing to tumor growth [62, 74]. (2) Mitochondrial function: TSPO is involved in mitochondrial function, including the regulation of mitochondrial membrane potential and the production of ROS. Altered mitochondrial function can contribute to changes in cellular metabolism and signaling pathways that promote tumor growth [75]. (3) Epigenetic regulation: TSPO expression can be regulated by epigenetic mechanisms, such as DNA methylation and histone modification. These epigenetic changes can contribute to the development of glioma tumors by modulating gene expression [76].
Regulation of TSPO expression in glioma cells
TSPO expression in glioma cells has garnered significant attention due to its involvement in tumor biology and neuroinflammation [59]. Abnormal TSPO expression has been implicated in the pathophysiology of gliomas, with studies indicating that high levels of TSPO correlate with tumor aggressiveness and a poor prognosis [51, 59]. This upregulation is often associated with the metabolic demands of rapidly proliferating tumor cells, as TSPO is involved in the regulation of mitochondrial functions essential for energy production and cellular metabolism [77].
There are mechanisms that regulate TSPO expression in gliomas: transcriptional activation, epigenetic regulation, and post-transcriptional modifications. Transcription factors such as NF-κB, SP1 (specific protein 1), and AP-1 (activator protein 1) have been shown to bind to the TSPO promoter in response to inflammatory cytokines, thereby enhancing its expression. Additionally, the hypoxic tumor microenvironment commonly found in gliomas can activate signaling pathways like HIF-1α (hypoxia-inducible factor-1alpha), further promoting TSPO upregulation [78]. Epigenetic regulation regulates TSPO expression by epigenetic modifications such as DNA methylation and histone modification. In glioma cells, DNA methylation and histone H3 lysine 27 trimethylation (H3K27me3) can repress TSPO expression, while histone H3 lysine 4 trimethylation (H3K4me3) can activate it [79]. The expression of TSPO is also influenced by post-transcriptional mechanisms, including microRNAs that can either promote or inhibit its expression, thus adding an additional layer of complexity to the regulation of TSPO in glioma pathology [80]. Post-translational regulation: TSPO expression can be regulated post-translationally by various mechanisms, including protein degradation, phosphorylation, and ubiquitination. In glioma cells, the ubiquitin-proteasome pathway can regulate TSPO stability and degradation [81].
Furthermore, the interaction between glioma cells and the surrounding tumor microenvironment also plays a critical role in TSPO regulation. Components such as tumor-associated macrophages and astrocytes may secrete various factors that modulate TSPO expression in glioma cells, indicating that the regulation of TSPO is not solely an intrinsic property of the tumor cells but also a reflection of the dynamic interactions within the tumor niche [82]. Collectively, these regulatory mechanisms highlight the potential of TSPO as both a biomarker for glioma progression and a therapeutic target, warranting further investigation into its role in tumor metabolism and microenvironmental interactions [83].
TSPO as a potential therapeutic target in glioma treatment
Current therapies targeting TSPO in gliomas
TSPO is overexpressed in various cancer types, including glioma, and targeting TSPO has been shown to be a promising therapeutic strategy for glioma treatment [51]. Some of the current therapies targeting TSPO in gliomas include:
Many PET probes for TSPO imaging have been developed, and several [11C] and [18F] labeled TSPO ligands have been evaluated in the human brain, such as [18F]FEPPA ([¹⁸F]N-(2-(2-fluoroethoxy)benzyl)-N-(4-phenoxypyridin-3-yl)acetamide), [18F]PBR-28 (N-(2-[¹⁸F]fluoroethyl)-N-(4-phenoxypyridin-3-yl)acetamide), and many others [57, 58, 84, 85]. These radioligands bind specifically to TSPO and allow for the detection of TSPO-expressing tumors. For instance, [11C]PK11195 ([11C]N-butan-2-yl-1-(2-chlorophenyl)-N-methylisoquinoline-3-carboxamide), [11C]PBR-28 (N-(2-[¹¹C]methoxybenzyl)-N-(4-phenoxypyridin-3-yl)acetamide), [18F]DPA-714 ([18F]N,N-diethyl-2-(2-(4-(2-fluoroethoxy)phenyl)-5,7-dimethylpyrazolo[1,5-a]pyrimidin-3-yl)acetamide), and others are used to image TSPO-positive glioblastoma tumors [51, 58, 86].
TSPO-based PET imaging using radioligands like [18F]FEPPA, [18F]DPA-714, [18F]PBR111 (2-(6-chloro-2-(4-(3-[¹⁸F]fluoropropoxy)phenyl)imidazo[1,2-a]pyridin-3-yl)-N,N-diethylacetamide), and others have been used to detect TSPO-expressing gliomas and monitor treatment response [58, 87].
TSPO-targeted immunotherapy: the TSPO has emerged as a crucial modulator of the immune microenvironment in gliomas, influencing various immune cell types such as T-cells, B-cells, and regulatory T-cells (Tregs). TSPO is highly expressed in glioma-associated microglia and macrophages [TAMs (tumor-associated microglia/macrophages)], contributing to immunosuppressive mechanisms that favor tumor survival and immune evasion. Specifically, TSPO expression in TAMs can facilitate mitochondrial function and oxidative stress regulation, affecting immune signaling pathways [63, 88]. This immunosuppressive environment is characterized by the promotion of Treg expansion, which inhibits the activation of cytotoxic CD8+ T-cells, hampering their ability to target and eliminate tumor cells [88, 89]. Moreover, TSPO is implicated in the alteration of B-cell activity within the glioma microenvironment. TSPO expression in infiltrating B-cells has been associated with a pro-tumorigenic cytokine profile, which further suppresses anti-tumor immune responses, highlighting the multifaceted role of TSPO in shaping the glioma immune landscape [90].
Immunotherapy: targeting TSPO presents a promising avenue for reversing the immune-osuppressive effects observed in gliomas. TSPO ligands, such as PK11195 and XBD173 (N-benzyl-N-ethyl-2-(7-methyl-8-oxo-2-phenylpurin-9-yl)acetamide), have demonstrated the capacity to reprogram TAMs toward a pro-inflammatory phenotype, thereby enhancing the efficacy of immune checkpoint inhibitors (ICIs) [91]. Additionally, combining TSPO-targeted therapies with strategies like chimeric antigen receptor T-cell (CAR-T) therapy could further improve T-cell infiltration and persistence within the glioma microenvironment, potentially overcoming barriers to effective anti-tumor immunity [92]. While the potential benefits of TSPO modulation in immunotherapy are significant, challenges such as off-target effects and the heterogeneity of gliomas must be critically addressed to optimize treatment outcomes.
TSPO-directed monoclonal antibodies (mAbs) represent a promising glioma immunotherapy strategy by inducing antibody-dependent cellular cytotoxicity (ADCC) and immune-mediated tumor clearance [93]. TSPO is overexpressed in glioma cells and TAMs, making it a selective immunotherapeutic target [94]. Upon binding to TSPO-expressing cells, TSPO-specific mAbs, such as [123I]GF3 ([123I]-N-(2-iodophenyl)-N-(4-methoxyphenyl)-1,2,3,4-tetrahydroisoquinoline-1-carboxamide), recruit natural killer (NK) cells through their Fcγ receptors, leading to the release of perforins and granzymes, which cause glioma cell apoptosis [95]. Additionally, TSPO-mAbs can enhance macrophage-mediated phagocytosis and activate cytotoxic T-cell responses, further promoting tumor elimination [96]. Preclinical studies demonstrated that anti-TSPO mAbs, such as 8D7, significantly reduce glioblastoma growth by modulating the tumor microenvironment and suppressing glioma proliferation [97]. However, challenges such as limited blood-brain barrier (BBB) permeability and glioma immune evasion mechanisms may affect therapeutic efficacy [94]. Current strategies, including nanoparticle-conjugated TSPO-mAbs and dual-targeting approaches combining TSPO-mAbs with PD-1 (programmed cell death-1) inhibitors, are being explored to enhance glioma immunotherapy [97].
TSPO-targeted photodynamic therapy (PDT): PDT uses light to activate a photosensitizer that targets TSPO-positive cells. Studies have shown that PDT can be effective in killing TSPO-positive glioma cells [98]. Targeting TSPO is a promising therapeutic strategy for glioma treatment, with various approaches being investigated, including radioligands, PET imaging, chemotherapy, immunotherapy, and PDT.
TSPO-targeted therapies are shown in Table 3, highlighting their merits, mechanisms of action (MOA), and clinical uses.
TSPO-targeted therapies and imaging in gliomas
Therapy | Merits | MOA | Clinical uses | Reference(s) |
---|---|---|---|---|
[11C]PK11195 | The high specificity for TSPO allows for tumor detection. | Binds to TSPO in the outer mitochondrial membrane, enabling PET imaging. | Imaging of TSPO-positive glioblastoma. | [86, 99] |
[18F]DPA-714 | It enhances imaging techniques and may reduce tumor growth. | TSPO antagonist, inhibiting tumor cell proliferation and inducing apoptosis. | Potential in clinical trials for therapy. | [36, 100, 101] |
[18F]FEPPA | High sensitivity and specificity for TSPO allow the monitoring of treatment responses. | Selectively binds to TSPO in the outer mitochondrial membrane, enabling PET imaging. | Detection of TSPO-expressing gliomas and monitoring therapies. | [102, 103] |
[18F]PBR111 | Non-invasive imaging and quantification of TSPO expression. | Binds to TSPO and enables PET imaging of tumor viability. | Used in glioma diagnosis and assessment of treatment efficacy. | [104] |
[11C]PBR-28 | Selectively eliminates TSPO-positive glioma cells. | Induces apoptosis in TSPO-expressing cells. | Targeted chemotherapy for treating TSPO-positive gliomas. | [102, 105] |
Ro5-4864 | Anti-proliferative effects in glioma cells promote apoptosis. | It inhibits TSPO, disrupting cellular metabolism and leading to cell death. | Preclinical studies focusing on glioma treatment. | [51, 106] |
[18F]GE180 | High binding affinity to TSPO and a better signal-to-noise ratio make it useful for distinguishing tumor types. | Binding to TSPO allows visualization of activated cells, specifically within tumor areas contributing to the glioma pathology. | Imaging of glioma to assess tumor viability and differentiate between types of gliomas based on TSPO expression. | [36, 66, 107] |
[11C]PBR-28: N-(2-[¹¹C]methoxybenzyl)-N-(4-phenoxypyridin-3-yl)acetamide; [11C]PK11195: [11C]N-butan-2-yl-1-(2-chlorophenyl)-N-methylisoquinoline-3-carboxamide; [18F]DPA-714: [18F]N,N-diethyl-2-(2-(4-(2-fluoroethoxy)phenyl)-5,7-dimethylpyrazolo[1,5-a]pyrimidin-3-yl)acetamide; [18F]FEPPA: [¹⁸F]N-(2-(2-fluoroethoxy)benzyl)-N-(4-phenoxypyridin-3-yl)acetamide; [18F]GE180: [18F]3-(2-fluoroethyl)-2-[(4-methoxyphenyl)thiomethyl]-8-methylquinazolin-4-one; [18F]PBR111: 2-(6-chloro-2-(4-(3-[¹⁸F]fluoropropoxy)phenyl)imidazo[1,2-a]pyridin-3-yl)-N,N-diethylacetamide; MOA: mechanisms of action; PET: positron emission tomography; Ro5-4868: 4'-chlorodiazepam; TSPO: translocator protein
Agents like TSPO antagonists are considered a direct approach to targeting TSPO, as they bind to the protein, potentially disrupting its function and leading to reduced cell survival and proliferation in glioma cells [36, 108]. In addition, inhibitors targeting downstream pathways influenced by TSPO, such as NF-κB and STAT3 (signal transducer and activator of transcription 3), offer a therapeutic strategy to modulate inflammation and immune evasion, which are critical for glioma progression [109]. By blocking these pathways, TSPO-associated pro-survival signaling can be suppressed, potentially slowing tumor growth [110–112]. ICIs represent another potential therapeutic avenue related to TSPO. Since TSPO is involved in immune modulation, targeting checkpoints [e.g., PD-1/PD-L1 (programmed cell death ligand-1)] in combination with TSPO modulation may enhance immune system activity against glioma cells, reducing the tumor’s ability to evade immune detection [113]. Furthermore, metabolic pathways influenced by TSPO offer a target to disrupt the metabolic adaptations glioma cells make for survival. Modulating these pathways could impair glioma cells’ energy production and biosynthesis, hindering tumor growth and survival [114]. Other key molecular targets and therapeutic strategies in glioma treatment have been shown in Table 4, highlighting molecular targets, pathways, effects on glioma cells, and potential therapeutic agents.
Molecular targets, pathways, effects on glioma cells, and potential therapeutic agents
Molecular target | Pathway | Effect on glioma cells | Potential therapeutic agents | Reference |
---|---|---|---|---|
TSPO | Mitochondrial function | Promotes cell survival, reduces apoptosis | TSPO ligands (e.g., [11C]PK11195) | [115] |
NF-κB | Inflammation | Enhanced by cytokine release | NF-κB inhibitors | [116] |
ROS | Oxidation stress | Increases oxidative damage and proliferation | Antioxidants (e.g., N-acetylcysteine) | [117] |
Cytochrome C | Apoptotic pathways | Reduced release lowers apoptosis | TSPO inhibitors | [118] |
[11C]PK11195: [11C]N-butan-2-yl-1-(2-chlorophenyl)-N-methylisoquinoline-3-carboxamide; NF-κB: nuclear factor kappa-light-chain-enhancer of activated B cell; ROS: reactive oxygen species; TSPO: translocator protein
Treatment selection with TSPO in glioma
Several studies have investigated the potential of TSPO as a biomarker for treatment selection in gliomas. These include: (1) TSPO expression is associated with poor prognosis, and this has been shown in a study published in the Journal of Neuro-Oncology that revealed that high TSPO expression was correlated with poor prognosis and shorter overall survival in patients with GBM [51]. This suggests that TSPO may be a useful biomarker for identifying patients with aggressive tumors. (2) A study published in the journal Cancer Research demonstrated that TSPO-targeting therapy using a small molecule inhibitor reduced tumor growth and increased survival in a mouse model of GBM. This suggests that TSPO may be a viable target for cancer therapy [51, 56, 65]. (3) TSPO expression predicts response to chemotherapy, and this has been shown in one study published in the Journal of Clinical Oncology, which found that high TSPO expression was associated with poor response to chemotherapy in patients with GBM. This suggests that TSPO may be used as a biomarker to predict response to chemotherapy. (4) TSPO-targeting therapy combined with chemotherapy has been studied and published in the Journal of Neuro-Oncology, which found that combining TSPO-targeting therapy with chemotherapy resulted in improved survival and reduced tumor growth in a mouse model of GBM.
Multimodal imaging approaches in combination with TSPO-targeted imaging in glioma
Multimodal imaging strategies enhance the diagnostic and therapeutic value of TSPO-targeted imaging by providing complementary information on tumor metabolism, vascularity, and molecular characteristics. In glioma, TSPO PET using radiotracers like [11C]PK11195, [18F]DPA-714, and [18F]GE180 ([18F]3-(2-fluoroethyl)-2-[(4-methoxyphenyl)thiomethyl]-8-methylquinazolin-4-one) can be combined with various imaging modalities to improve sensitivity and specificity [85]. MRI, including contrast-enhanced MRI, DWI, and DSC perfusion MRI, is commonly used alongside TSPO PET to assess tumor size, vascular permeability, and treatment response [15]. MRS provides metabolic profiling by detecting glioma-associated metabolites such as choline, N-acetyl aspartate, and lactate, complementing TSPO imaging in differentiating tumor progression from pseudoprogression [16]. Additionally, FDG-PET can assess glioma glucose metabolism, while amino acid PET (e.g., [18F]FET, [11C]MET PET) improves tumor delineation beyond TSPO PET alone [119]. Photoacoustic imaging (PAI) and optical imaging techniques are emerging as adjuncts to TSPO-based imaging for intraoperative tumor visualization [120]. These multimodal approaches enhance glioma characterization, improving diagnostic accuracy, treatment monitoring, and surgical planning [121].
Potential future strategies for targeting TSPO in glioma treatment
Some of the potential future strategies for targeting TSPO in glioma treatment include (1) TSPO-based imaging: TSPO is overexpressed in glioma cells, making it a possible target for imaging modalities such as PET. Studies have shown that TSPO-based PET imaging can detect glioma recurrence and monitor treatment response [36, 122]. (2) TSPO-targeting therapeutics: several small-molecule compounds have been developed to target TSPO, including ligands that bind to the TSPO receptor and inhibit its activity. For example, PK11195, a well-known TSPO ligand, has been shown to inhibit glioma cell proliferation and induce apoptosis [62, 123]. (3) TSPO-targeting nanoparticles, nanoparticle-based delivery systems, can be designed to target TSPO-expressing glioma cells, allowing for enhanced delivery of chemotherapeutic agents or other therapeutic molecules. For example, TSPO-targeted liposomes have been shown to deliver doxorubicin to glioma cells selectively [124]. (4) TSPO-based gene therapy and the gene therapy approaches that target TSPO have been explored as a means to deliver therapeutic genes to glioma cells. For example, a study demonstrated that a TSPO-targeted adenovirus vector could efficiently deliver the suicide gene herpes simplex virus thymidine kinase (HSV-tk) to glioma cells [125–127]. (5) Combination therapy: targeting TSPO in combination with other therapeutic agents may enhance treatment efficacy and reduce side effects. For example, a study combining PK11195 with temozolomide showed enhanced anti-glioma activity compared to either agent alone [128, 129]. (6) TSPO-targeting immunotherapy: ICIs have revolutionized cancer treatment, but their efficacy in glioma is limited due to the immunosuppressive tumor microenvironment. Targeting TSPO may enhance the activity of immunotherapies by increasing immune cell infiltration and activation within the tumor. For example, a study showed that PK11195 increased the expression of PD-1 on T-cells and enhanced their anti-tumor activity [125]. (7) TSPO-targeting immunotoxins, in which immunotoxins are fusion proteins that combine an antibody targeting TSPO with a cytotoxin. These molecules have been shown to selectively kill TSPO-expressing glioma cells while sparing normal cells [125]. (8) Targeting TSPO in glioma therapy has been proposed as a potential therapeutic strategy for glioma treatment, and several approaches have been explored, including (i) TSPO inhibitors [130, 131]; (ii) TSPO-targeting antibodies [132]; (iii) TSPO siRNA (short interfering ribonucleic acid)-based therapy: siRNA targeting TSPO has been shown to inhibit glioma cell proliferation and survival [125, 131].
Challenges and opportunities in developing TSPO-targeted therapies
Challenges in developing TSPO-targeted therapies
TSPO heterogeneity: TSPO has multiple isoforms, which can lead to inconsistent results and challenges in identifying specific binding sites for therapeutic compounds [96].
TSPO expression: TSPO is expressed in various tissues, including the brain, liver, and immune cells, which can make it challenging to target specific tissues.
Selectivity: developing compounds that selectively target TSPO while avoiding off-target effects can be challenging [123].
Off-target binding, in which TSPO has been shown to bind to other proteins, including cluster of differentiation 11b (CD11b), can lead to off-target effects and potential side effects [133]. TSPO-targeted therapies face significant challenges due to off-target effects and lack of tissue specificity [134]. While TSPO is overexpressed in gliomas, it is also found in normal brain cells, activated microglia, peripheral immune cells, and mitochondrial membranes, leading to non-specific binding and potential toxicity [53]. This can result in unwanted neuroinflammation, mitochondrial dysfunction, and systemic side effects, limiting the therapeutic window [135]. Additionally, the heterogeneous expression of TSPO across different glioma subtypes reduces its reliability as a universal target [89]. To overcome these limitations, alternative glioma-targeting strategies are being developed. One promising approach is targeting glioma-specific antigens such as IL13Rα2 (interleukin-13 receptor alpha 2), EGFRvIII (epidermal growth factor receptor variant III), HER2 (human epidermal growth factor receptor 2), and GD2 (disialoganglioside), which are highly expressed in glioblastoma but absent in normal brain cells [136]. Nanoparticle-based drug delivery systems can also improve specificity by encapsulating therapies and releasing them selectively in the tumor microenvironment [137]. Furthermore, BBB-penetrating peptides and engineered exosomes offer innovative ways to deliver drugs directly to glioma cells while minimizing systemic exposure [138]. Future strategies include dual-targeting CAR-T cells that combine TSPO with glioma-specific antigens to enhance specificity while reducing off-target toxicity [139].
Opportunities in developing TSPO-targeted therapies
In immunomodulation, TSPO has been shown to play a role in immune cell function and activation. Targeting TSPO may offer a new approach to treating autoimmune diseases [59]. Additionally, in cancer therapy, TSPO has been implicated in cancer progression and metastasis. Targeting TSPO may offer a new therapeutic approach to cancer treatment [140–142]. Furthermore, in imaging applications, TSPO has been used as a biomarker for imaging neuroinflammation in gliomas, neurodegenerative diseases, and other diseases. Targeting TSPO may offer new opportunities for imaging applications. This allows for early detection and monitoring of tumor progression, and this can lead to more accurate diagnosis and treatment planning. Integrating TSPO-targeted agents with existing treatment modalities, such as chemotherapy or immunotherapy, could enhance therapeutic efficacy and improve clinical outcomes in patients with refractory tumors [125, 143].
Future implications and directions for research
Future implications
TSPO has been shown to be overexpressed in gliomas, making it a potential target for neuroimaging. A TSPO-based imaging agent could help diagnose gliomas earlier and more accurately, allowing for timely intervention and improved patient outcomes, leading to improved diagnosis [36]. In addition, by using TSPO as a biomarker, researchers can identify patients who are more likely to respond to specific treatments, such as chemotherapy or radiation therapy. This could lead to more effective treatment strategies and reduced side effects, leading to personalized treatment [67]. Furthermore, TSPO expression has been linked to glioma progression and aggressiveness. Monitoring TSPO levels using neuroimaging could help track disease progression and identify patients who may require more aggressive treatment, leading to disease progression monitoring [58].
Directions for research
Development of novel imaging agents: researchers need to develop new imaging agents that can specifically target TSPO and provide high-resolution images of gliomas. This could involve designing novel small molecules that bind to TSPO with high affinity [65]. Optimization of imaging protocols: to improve diagnostic accuracy and sensitivity, researchers need to optimize imaging protocols, including the choice of imaging modality, imaging sequence, and dosing regimen [144]. Additionally, to correlate with clinical outcomes to validate the use of TSPO as a biomarker, researchers need to correlate TSPO expression with clinical outcomes, such as patient survival and response to treatment [145]. Furthermore, investigating TSPO regulation involves understanding the regulation of TSPO expression in gliomas and is important for developing effective therapeutic strategies. Researchers need to investigate the molecular mechanisms underlying TSPO regulation and identify potential therapeutic targets as well as translation to clinical trials, in which the findings from preclinical studies should be translated to clinical trials to test the efficacy and safety of TSPO-based imaging agents in human patients [146].
Conclusions
Overexpression of TSPO in glioma cells, particularly high-grade tumors, shows a strong link to tumor aggressiveness and poor prognosis. High-specificity and high-sensitivity TSPO-targeted imaging agents are promising tools for glioma diagnosis and progression monitoring. Optimizing and validating TSPO-based imaging protocols in clinical settings will improve diagnostic precision and patient outcomes. Investigating multimodal imaging approaches that combine TSPO-targeted imaging with other imaging techniques will enhance glioma detection accuracy and therapeutic monitoring.
Abbreviations
[11C]MET: | L-[methyl-¹¹C]methionine |
[11C]PK11195: | [11C]N-butan-2-yl-1-(2-chlorophenyl)-N-methylisoquinoline-3-carboxamide |
[18F]DPA-714: | [18F]N,N-diethyl-2-(2-(4-(2-fluoroethoxy)phenyl)-5,7-dimethylpyrazolo[1,5-a]pyrimidin-3-yl)acetamide |
[18F]FDG: | 2-[¹⁸F]fluoro-2-deoxy-D-glucose |
[18F]FEPPA: | [¹⁸F]N-(2-(2-fluoroethoxy)benzyl)-N-(4-phenoxypyridin-3-yl)acetamide |
[18F]FET: | O-(2-[18F]fluoroethyl)-L-tyrosine |
[18F]FLT: | [18F]3'-deoxy-3'-fluorothymidine |
BBB: | blood-brain barrier |
CAR-T: | chimeric antigen receptor T-cell |
CDKs: | cyclin-dependent kinases |
CT: | computed tomography |
DSC: | dynamic susceptibility contrast |
DWI: | diffusion-weighted imaging |
EMT: | epithelial-mesenchymal transition |
GBM: | glioblastoma multiforme |
ICIs: | immune checkpoint inhibitors |
MAbs: | monoclonal antibodies |
MRI: | magnetic resonance imaging |
MRS: | magnetic resonance spectroscopy |
NF-κB: | nuclear factor kappa-light-chain-enhancer of activated B cells |
PD-1: | programmed cell death-1 |
PDT: | photodynamic therapy |
PET: | positron emission tomography |
PI3K/AKT/mTOR: | phosphoinositide 3-kinase/protein kinase B/mammalian target of rapamycin |
PSMA: | prostate-specific membrane antigen |
ROS: | reactive oxygen species |
siRNA: | short interfering ribonucleic acid |
SPECT: | single photon emission computed tomography |
TAMs: | tumor-associated microglia/macrophages |
Tregs: | regulatory T-cells |
TSPO: | translocator protein |
Declarations
Acknowledgments
We acknowledge the support of the Department of Nuclear Medicine at Nanjing First Hospital.
Author contributions
JM: Writing—original draft. BL and JW: Writing—review & editing. FW: Conceptualization, Investigation, Writing—review & editing, Funding acquisition. YY: Conceptualization, Investigation, Writing—review & editing.
Conflicts of interest
The authors declare that they have no conflicts of interest.
Ethical approval
Not applicable.
Consent to participate
Not applicable.
Consent to publication
Not applicable.
Availability of data and materials
Not applicable.
Funding
The National Key Research and Development Program of China [2022YFC2406900] supports this work. The key subjects of nuclear medicine: Jiangsu Provincial Institute of Medical Sciences [JSDW202247]. Jiangsu Provincial Medical Key Discipline Cultivation Unit [JSDW202247]. Nanjing International/Hong Kong, Macao, and Taiwan Science and Technology Cooperation Program Project [202308005]. The National Natural Science Foundation of China [82301609], China Postdoctoral Science Foundation [2022M711666], Natural Science Foundation of Jiangsu Province [BK20220196], the International Joint Research and Development Project of Nanjing [202201030], and the International Joint Research and Development Project of Nanjing [202308005]. The funders had no role in study design, data collection and analysis, decision to publish, or preparation of the manuscript.
Copyright
© The Author(s) 2025.
Publisher’s note
Open Exploration maintains a neutral stance on jurisdictional claims in published institutional affiliations and maps. All opinions expressed in this article are the personal views of the author(s) and do not represent the stance of the editorial team or the publisher.