Abstract
Aim:
Amyotrophic lateral sclerosis (ALS) is a progressive disease of unknown etiology, characterized by degeneration of motoneurons and skeletal muscle strength decline that progressively evolves to respiratory failure and death. A key point in the therapeutic approach is to understand the pathological processes associated with disease evolution. In spite of intensive research on the molecular/cellular mechanisms involved in ALS initiation and progression disease etiology, unfortunately, poorly understood and there is no efficient specific/decisive treatment for ALS patients. The aims of the present study are to identify specific factors in the cerebrospinal fluid (CSF) of ALS patients and to test their potential relevance to the etiology of this disease.
Methods:
Peptides were identified by liquid chromatography tandem mass spectrometry (LC-MS/MS). Motor activity of mice was tested by the Rota-rod test and peptide-induced inflammation was assessed by induction nitric oxide synthase activity in BV2 microglia cells.
Results:
Analysis of CSF samples of ALS patients (n = 15) detected two peptides, C-terminal fragments of transthyretin and osteopontin, which were absent in a control group (n = 15). In addition to being potential biomarker candidates, the relevancy of these peptides to the disease etiology was tested by assessing their effects on motor activity in mice and inflammation model in cell culture. Intranasal administration of the peptides reduced motor activity in the Rota-rod test and activated lipopolysaccharide-induced inflammation in BV2 microglia cells.
Conclusions:
These findings suggest that during ALS onset and progression two potentially neurotoxic peptides are formed, released, or penetrated the central nervous system thus inducing neuroinflammation and neurodegeneration.
Keywords
Amyotrophic lateral sclerosis, peptides, neuroinflammation, motoneurons, transthyretin, osteopontinIntroduction
Amyotrophic lateral sclerosis (ALS) is a progressive disease characterized by degeneration of the cortical upper motoneurons and lower motor neurons in the brain stem and spinal cord [1, 2]. Consequently, skeletal muscle strength declines and progressively evolves to respiratory failure leading to death of most patients 3–5 years after diagnosis [2, 3]. The incidence of ALS ranges between 0.6 and 3.8 per 100, 000 person-years, and the prevalence between 3.1 and 8.4 per 100 ,000 person-years [4, 5]. The association between ALS incidence and geographic location is questionable and may partly be due to gene [chromosome 9 open reading frame 72 (C9orf72)] [6] and environmental risk factors [7–10]. Increasing evidence indicates that neuroinflammation mediated by microglial activation contributes to ALS pathogenesis [11, 12]. This is of particular interest due to the effect of environmental pollutants on microglia [13] and the role of these cells in ALS pathogenesis [14]. In spite of intensive research on the environmental factors and the molecular/cellular mechanisms involved in ALS initiation and progression disease etiology is unfortunately poorly understood and there is no efficient specific/decisive treatment for ALS patients [3].
Recent studies demonstrated that gut microbiome and impaired intestinal barrier integrity play an important role in the pathophysiology of neurological diseases such as Parkinson’s disease [15] and Alzheimer’s disease [16]. Gut permeability can be adversely affected by various toxic agents such as heavy metals [17, 18], pesticides [19], and mycotoxins [20]. This effect may be mediated by imbalanced gut flora (dysbiosis) that impairs intestinal functions and gut integrity [17, 18]. Consequently, bacterial toxins penetrate the intestinal wall into the blood stream reaching the blood-brain barrier (BBB) and adversely affecting the neuronal system [21].
Recent studies demonstrated increased circulatory lipopolysaccharide (LPS) levels in ALS patients and other neurodegenerative diseases [22, 23] indicating impaired gut integrity. Further support was obtained by the increased levels of the intestinal permeability indicator zonulin in ALS and, Alzheimer’s and Parkinson’s diseases [22]. The leaky gut and shifted profile of intestinal microbiome were also demonstrated in ALS animal model [24]. These studies indicate the involvement of gut integrity in the pathogenesis of ALS and other neurodegenerative diseases and may serve as tools for the future development of medical treatment. Even with the more sensitive Gold Coast criteria [25], ALS diagnosis is generally late, leading to a delay of about one year between the onset of symptoms and the diagnosis. The optimal solution for this problem would be the development of biomarkers for early and robust diagnosis of the disease. Moreover, it is now evident that the success of clinical trials in ALS is critically dependent on the use of appropriate biomarkers, i.e., sensitive and accurate measures of disease progression [26] and response to trial therapy [27]. Several candidate biomarkers for ALS have emerged, including neurophysiological and neuroimaging measures and biofluids such as kynurenine, tryptophan, quinolinic acid [28], and the most promising prognostic marker neurofilament light chain [29, 30], but so far lack consensus and uncomplete knowledge hampered their use in clinical practice. Therefore, specific biomarkers are needed for each of the targeted mechanisms, to evaluate the contributions of individual therapies [27, 31] for early diagnosis, and to gain insight into the etiological and pathogenetic mechanisms underlying this disease.
In the present study, two peptides have been identified in the cerebrospinal fluid (CSF) of ALS patients that may serve as potential biomarker candidates. The involvement of these peptides in ALS etiology was tested by their ability to induce clinical signs in experimental animals and inflammatory response in microglia cells.
Materials and methods
Subjects and methods
Study participants
Two groups of ALS patients and controls residing in the Emilia-Romagna region in northern Italy were recruited. The ALS group was composed of 58 individuals diagnosed with definite or probable ALS (revised El Escorial criteria) at the ALS Centre of the Modena University Neurological Department from 2001 to 2011. They underwent lumbar puncture inter alia, primarily for diagnostic procedures; and at least 1 mL CSF was available for the present study (15 patients were selected, 10 males and 5 females). The mean age at disease onset was 52 years (range 31–62 years), 13 most of the patients (n = 13) presented with spinal onset, and 2 with bulbar onset ALS. Neither dementia nor extrapyramidal signs were presented in the ALS patients. The distribution of phenotypes was as follows: 8 classic, 3 flail, 2 bulbar, and 2 upper motor neuron predominant phenotype. The mean disease duration from onset to death or last observation was 67 months. Riluzole treatment was started in all the patients after CSF sample withdrawal. Genetic screening was carried out in 10 of the patients including all the major genes that are implicated in ALS [superoxide dismutase 1 (SOD1), C9orf72, fused in sarcoma (FUS), TAR DNA-binding protein 43 (TDP43)] with none testing positive. Due to a lack of available blood samples genetic analysis could not be performed in the remaining patients.
The control group consisted of residents in the Emilia-Romagna region who were admitted to the same department between 2001 and 2011 and underwent lumbar puncture because of suspected but later on unconfirmed neurological disease. Headache and paresthesia were the main signs that led to the neurological examination and lumbar puncture. Fifteen (9 males and 6 females) of these subjects were selected by age-matching (± 10 years) 1:1 to ALS patients. The 15 age-matched controls had a mean age of 54 years (range 32–71 years).
Sample collection
CSF was collected by lumbar puncture from each participant and immediately stored at –80°C in polypropylene tubes. A 1 mL aliquot was transported by air courier deep frozen in dry ice to the Institute of Drug Research (U. W.’s laboratory) at the Hebrew University of Jerusalem and kept continuously frozen until use.
Isolation and identification of CSF peptides
Samples
Fifteen CSF specimens of ALS patients were randomly divided into 3 groups of 5 pooled specimens (6 µL each specimen, a total of 30 µL per group). The same procedure was carried out with the 15 control specimens. Peptide analysis of both groups (3 samples each) was carried out by liquid chromatography tandem mass spectrometry (LC-MS/MS).
LC-MS/MS
The peptides were extracted in 8 mol/L urea, 100 mmol/L ammonium bicarbonate, and sonicated. They were reduced with 2.8 mmol/L dithiothreitol for 30 min at 60°C, and modified with 8.8 mmol/L iodoacetamide in 100 mmol/L ammonium bicarbonate in the dark for 30 min at room temperature. The peptides were desalted by C18 tips (Harvard Bioscience Inc.) dried and re-suspended in 0.1% formic acid. All peptides were analyzed by LC-MS using a Q ExactiveTM Plus mass spectrometer (Thermo Fisher Scientific Inc.) fitted with a capillary high performance liquid chromatography (EASY-nLC 1000, Thermo Fisher Scientific Inc.). The peptides were loaded onto a C18 trap column (0.3 mm × 5 mm, LC-Packings) connected on-line to a homemade capillary column (20 cm, 75 μm inner diameter) packed with ReproSil C18-Aqua (Dr Maisch GmbH, Germany) in solvent A (0.1% formic acid in water). The peptide mixture was resolved with a (5% to 28%) linear gradient of solvent B (95% acetonitrile with 0.1% formic acid) for 180 min followed by gradient of 10 min gradient of 28% to 95% and 25 min at 95% acetonitrile with 0.1% formic acid in water at flow rates of 0.15 μL/min. Mass spectrometry (MS) was performed in a positive mode [mass-to-charge ratio (m/z) 300–1,800, resolution 70,000 ppi] using repetitively full MS scan followed by collision induces dissociation [higher-energy collisional dissociation (HCD), at 25 normalized collision energy] of the 10 most dominant ions (> 1 charges) selected from the first MS scan. The automatic gain control (AGC) settings were 3 × 106 for the full MS and 1 × 105 for the MS/MS scans. The intensity threshold for triggering MS/MS analysis was 1 × 104. A dynamic exclusion list was enabled with an exclusion duration of 20 s.
Data analysis
MS data were analyzed by the MaxQuant software 1.5.1.2 (https://www.maxquant.org/) for peak picking identification and quantitation using the Andromeda search engine, searching against the human Uniprot database with a mass tolerance of 20 ppm for the precursor masses and 20 ppm for the fragment ions. Oxidation on methionine proline and lysine, and protein N-terminus acetylation were accepted as variable modifications, and carbamidomethyl on cysteine was accepted as static modifications. Minimal peptide length was set to six amino acids and a maximum of two miscleavages was allowed. Peptide- and protein-level false discovery rates were filtered to 1% using the target-decoy strategy. Protein table was filtered to eliminate the identifications from the reverse database, and common contaminants and single peptide identifications. The data were quantified by label-free analysis using the same software, based on extracted ion currents of peptides enabling quantitation from each LC-MS run for each peptide identified in any of the samples.
Experimental animals
Mice were supplied by Harlan Laboratories Breeding Center, Ein Karem, Jerusalem, Israel. Following an acclimation period of at least 7 days to ensure their suitability for the study, animals were randomly assigned to experimental groups. The animals were kept in a species pathogen-free rodent facility with environmental conditions set to a temperature of 20°C ± 2°C, a humidity of 30–70% (monitored daily), and a 12-hour light/12-hour dark cycle. The animals were housed in filter-covered polypropylene cages with solid bottoms and covered with wood shavings as bedding material. Animals were provided ad libitum access to a commercial mouse diet and drinking water was supplied to each cage via propylene bottles with stainless steel sipper tubes.
Peptide formulation and intranasal administration
Formulation
A nano-sized emulsion (so-called microemulsion) formulation was made by combining an aqueous (water) phase and an oily phase at a 4:1 ratio to form an isotropic liquid. The oily phase was composed of glyceryl oleate and Labrasol (caprylocaproyl macrogol-8 glycerides, the surfactants) at 1:3 ratio, 13% isopropyl palmitate (oil), and propylene carbonate (co-surfactant) at a co-surfactant/surfactant’s ratio of 1:5. A volume of 0.25 mL of the oily phase was mixed with a 1 mL peptide solution (1.25 mg/mL water) giving a concentration of 1 mg/mL.
Animal treatment
Male ICR mice (25–30 g) were intranasally administered with NER2 peptide (see the sequence in the Results section) at a dose of 2 µg (2 µL)/nostril, a total of 4 µg per animal, 5 times a week. On day 34 NER2 was replaced by NER3 (see the sequence in the Results section) for the rest of the study. The control group was administered with the vehicle at the same volume. The Rota-rod test was performed once a week (n = 4 for each group).
Rota-rod test
Motor coordination, strength, and balance were assessed using a rotarod (Rota-Rod treadmills ENV-577M five station mouse, Med Associates Inc.). Animals were placed onto the cylinder at a mode of 44 rounds per minute and the time (min) until fall was recorded for each animal. Mice were trained on the instrument 2 weeks prior to onset of peptide treatment. The Rota-rod test was performed before the daily intranasal injection. In the end of experiment the animals were euthanized by overdose of isoflurane inhalation.
LPS-induced inflammation in BV2 cells
BV2 microglial cells were seeded in 96 well plates (6 × 104 cells/200 µL medium) and incubated for 24 h. Peptides (100 ng/mL) or LPS (1 µg/mL) were added to the cells and nitric oxide (NO) as nitrite was determined in the medium after 24 h by Griess reagent (Sigma-Aldrich®) according to manufacturer instructions. Briefly, 10 µL Griess reagent I and 10 µL Griess reagent II were added to the tested medium (or standard nitrite solution) diluted with appropriate volume of nitrite assay buffer in a total volume of 200 µL in a 96 well plate. Absorbance was measured after 10 min using a filter of 540 nm.
Statistical evaluation
Student’s t test was used for statistical evaluation of the differences between the treated and control groups.
Results
Identification of peptides in CSF of ALS patients
Analysis of CSF samples identified two peptides in the CSF of ALS patients while no detectable amounts were found in the control group. The peptides are carboxy-terminal fragments of transthyretin (termed NER2) and osteopontin (NER3). Their sequences are as follows:
NER2: ALLSPYSYSTTAVVTNPKE (transthyretin 129–147 position, molecular weight 2,040.0 Dalton);
NER3: ISHELDSASSEVN (osteopontin 302–314 position, molecular weight 1,386.6 Dalton).
Both NER2 and NER3 may serve as potential ALS biomarkers for disease diagnosis. Nevertheless, these peptides may also be considered as contributing factors in ALS etiology. For testing the potential role of NER2 and NER3 in disease pathogenesis we carried out primary studies aimed to test their effect on motor activity in mice.
Pharmacological activities of NER2 and NER3
Effect of peptides on motor activity
Motor activity was monitored by the Rota-rod test. Impaired motor functions were observed after intranasal administrations of NER2 and NER3 using nanosized formulations for targeting the brain. A marked reduction in motor functions (expressed by the time to fall down from the rotating cylinder) was observed in the peptide-treated group as compared to the control animals, which retained their normal activity during the 167 days of the experiment (Figure 1).
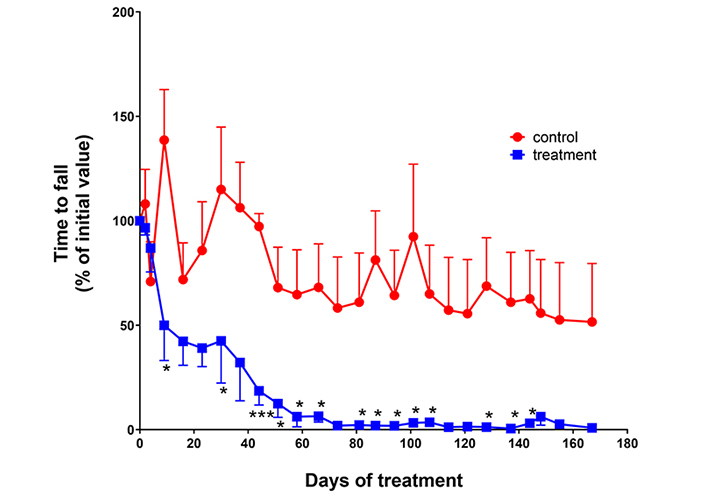
Effect of peptides on motor activity. Male ICR mice (25–30 g) were intranasally administered with NER2 peptide (nanosized formulation) at a dose of 2 µg dissolved in 2 µL/nostril, total of 4 μg/2 nostrils) 5 times a week. On day 34, NER2 was replaced by NER3 for the rest of study. Control group was administered with the unloaded vehicle at the same volume. Rota-rod test was performed once a week. Animals were trained on the instrument 2 weeks prior to onset of peptide treatment. n = 4 for each group. *** P = 0.0001; * P < 0.05
Effect of peptides on inflammatory response in microglia cells
Incubation of microglia cells with the endotoxin LPS caused activation of inflammatory processes including induction of NO synthase (iNOS type) expressed by the production of NO which spontaneously converted into nitrite in the culture medium. Addition of NER2 or NER3 to the endotoxin caused a slight but statistically significant increase of 14.7% and 10%, respectively, in NO production as compared to LPS only (Figure 2). Addition of the peptides without LPS resulted in slight but not significant activation of the inflammatory response.
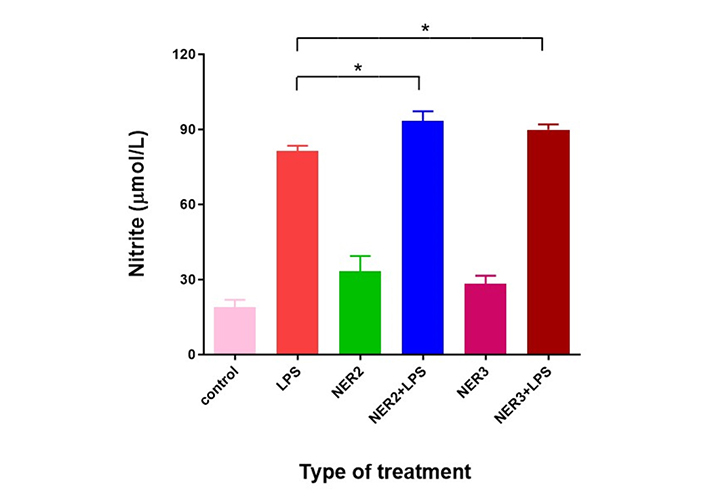
Effect of LPS combined with NER2 and NER3 peptides on BV2 microglia cells. BV2 cells were seeded in 96 well plate (6 × 104 mL, volume of 200 µL/well). LPS (1 µg/mL) and peptides (100 ng/mL each) were added after 24 h. NO production expressed by nitrite levels was determined 96 h after LPS and peptide addition. n = 5 for each experimental group. * P < 0.05
Discussion
In the present study, two peptides in the CSF of ALS patients have been discovered, NER2 and NER3, which are fragments of transthyretin and osteopontin, respectively. Transthyretin is mainly synthetized in the liver [32] and the choroid plexus, in which most of the CSF is produced and secreted [33]. NER2 might be a product of the abnormal proteolytic breakdown of transthyretin in the choroid plexus of ALS patients, a process that does not occur in healthy individuals. Osteopontin is expressed by various tissues and organs including the central nervous system (CNS) and preferentially in the primary motor cortex inside the cell bodies of neurons [34]. This protein takes part in ALS neurodegeneration [35]; it induces matrix metalloproteinase 9 (MMP9), the proteolytic activity of which contributes to neuronal cell destruction and especially axonal degeneration [36]. This is in line with recent studies showing the upregulation of osteopontin in ALS and other neurodegenerative diseases [37, 38]. Moreover, increased levels of plasma osteopontin at disease diagnosis predicted shorter survival in ALS patients [39]. MMP9 cleaves osteopontin [40] while one of the specific cleavage sites is between arginine 301 and isoleucine 302 [41] to form NER3. This concept of abnormal in situ proteolytic degradation leading to NER2 and NER3 formation needs further elucidation.
The neurotoxic effect of NER2 and NER3 is reflected by the impaired motor activity observed in the peptide-treated mice. The presence of their protein precursors, namely transthyretin and osteopontin in the CNS and their cleavage to neurotoxic peptides may potentially be involved in ALS pathogenesis. The mechanism of action of the neurotoxic activity may be direct cytotoxicity to neuronal cells (unpublished results) causing necrotic/apoptotic effect and/or via LPS coactivation of microglia and elicitation of inflammatory response resulting in neuronal damage. Future studies are needed to explore the mechanism of action of these neurotoxic peptides.
Previous studies showed detectable amounts of NER2 in the plasma of healthy humans [42, 43]. Plasma analysis carried out in our lab identified NER3 peptide in healthy as well as multiple sclerosis patients (unpublished results). Like many other substances, these circulatory peptides do not penetrate the BBB due to its powerful control on the influx and efflux of substances that maintain the steady-state environment of the CNS [44]. However, in ALS and other neurodegenerative diseases the BBB filtrating capacity is impaired [45] enabling NER2 and NER3 penetration into the brain.
BBB integrity can be adversely affected by a variety of toxins of which LPS is one of the important factors relevant to neurodegeneration. Among the targets directly disrupted by this endotoxin are the tight junctions and adherence junctions located among the brain endothelial cells; LPS-induced MMP9 activation further contributes to the degradation of tight junctions and thereby elevates BBB permeability [44].
Plasma analyses showed elevated levels of LPS in ALS and other neurodegenerative diseases [22, 23]. Interestingly, the intestinal permeability indicator zonulin was also increased in ALS and other neurodegenerative disorders like Alzheimer’s and Parkinson’s diseases and was correlated with LPS plasma levels [22]. These data provide evidence for intestinal hyperpermeability in ALS and other neurodegenerative disorders which may be responsible for the high circulating levels of LPS [22]. This endotoxin disrupts the BBB and enables penetration of NER2, NER3, and the LPS into the CNS, causing neuroinflammation and neurodegeneration (see Figure 3). Repairing leaky gut may reduce plasma LPS levels, improve BBB functions, reduce NER2/NER3 peptides and LPS infiltration into the CNS, and slow down disease progression [46]. This proposal for a mechanism of pathogenesis needs future investigation alongside experimental and clinical confirmation.
Abbreviations
ALS: | amyotrophic lateral sclerosis |
BBB: | blood-brain barrier |
CNS: | central nervous system |
CSF: | cerebrospinal fluid |
LC-MS/MS: | liquid chromatography tandem mass spectrometry |
LPS: | lipopolysaccharide |
MMP9: | matrix metalloproteinase 9 |
MS: | mass spectrometry |
NO: | nitric oxide |
Declarations
Acknowledgments
Murine BV2 microglia were kindly provided by Professor Rosario Donato (Department of Experimental Medicine and Biochemical Sciences, University of Perugia, Italy). The authors thank Professor Sigal Fleisher-Berkovich for her technical assistance and Dr. Tamar Ziv for peptide analyses.
Author contributions
UW: Conceptualization, Supervision, Writing—original draft, Writing—review & editing. AS: Data curation, Methodology, Writing—original draft, Writing—review & editing. MV: Formal analysis, Writing—review & editing. JM: Data curation, Formal analysis, Writing—review & editing. BB: Methodology, Project administration, Formal analysis, Writing—review & editing. EP: Methodology, Data curation, Formal analysis, Writing—review & editing. YF: Conceptualization, Methodology, Writing—original draft, Writing—review & editing. All authors read and approved the final manuscript.
Conflicts of interest
The authors declare that they have no conflicts of interest.
Ethical approval
The present study (including all procedures, maintenance and treatment of the animals) was approved by the Institutional Animal Care Committee of the Hebrew University of Jerusalem, Israel, No. NS-16-14983-4. The Hebrew University of Jerusalem is an AAALAC International accredited institute. The CSF specimens for the present study was approved by the Modena Ethical Committee.
Consent to participate
Informed consent to publication was obtained from relevant participants.
Consent to publication
Not applicable.
Availability of data and materials
Data are available upon request from the corresponding author.
Funding
Not applicable.
Copyright
© The Author(s) 2023.