Abstract
Aim:
In this study, the finite elements analysis (FEA) was performed on an intramedullary (IM) pin to be used in the canine femur. The 03 different biomaterials [17-4-precipitated hardened (PH)-stainless steel (SS), nickel alloys (Ni)-625, titanium alloys (Ti)-6Al-4V] were selected for comparative FEA. In-vitro analysis was also performed in simulated body fluid (SBF) on selected biomaterials for possible application in the canine femur.
Methods:
FEA was performed on 03 different biomaterials (17-4-PH-SS, Ni-625, and Ti-6Al-4V) based on Von-Mises criteria (at an applied load of 1,500 N, cell type: tetrahedron, grit size: 0.15 mm, number of nodes: 213,989 and elements: 145,012). The distal end of the IM pin was fixed, and the load was applied to the proximal end. In-vitro analysis was performed (on a potentiostat setup) to establish the corrosion rate of various biomaterials (17-4-PH-SS, Ni-625, and Ti-6Al-4V).
Results:
The results of FEA show Ni-625 absorbed the maximum Von-Mises stress in the case of tensile and compression loading (104.12 MPa). In the case of torsion loading, the maximum Von-Mises stress was absorbed by 17-4-PH-SS (63.331 MPa). The maximum Von-Mises elastic strain (0.00093473) was observed for Ti-6Al-4V while tensile and compression and minimum deformation (0.013869 mm) in tensile loading.
Conclusions:
Based on this study, the maximum safety factor against failure (N) [ratio of 0.2% of yield strength (σy) to the Von-Mises stress (σv)] was observed as 10.75, 11.38, and 15.89, respectively, for tensile, compression, and torsional loading in the case of Ti-6Al-4V. Also, the better biocompatible material for the orthopaedic implant application based on the corrosion result is Ti-6Al-4V due to a lower corrosion rate (2.63211 × 10–10 mm/year) in comparison to 17-4-PH-SS and Ni-625. Overall, the Ti-6Al-4V is a better material than 17-4-PH-SS and Ni-625 for the intended application.
Keywords
Intramedullary pin, laser powder bed fusion, biomaterials, implantIntroduction
Layering metal materials to create three-dimensional (3D) structures is known as metal 3D printing/additive manufacturing (AM) [1]. In contrast to conventional subtractive manufacturing techniques such as milling, cutting, or moulding, metal 3D printing uses digital models to create products layer by layer. A few benefits of this technique include its capacity to build complicated and intricate shapes [2], decreased material waste, and design freedom [2, 3]. Laser powder bed fusion is one of the most widely used 3D printing methods for metal [3]. This method involves spreading a thin layer (≈ 30 µm) of metal powder on a build platform, then selectively melting and fusing the powder following the cross-section of the 3D model using a laser [4]. Layer by layer, this procedure is continued until the complete item is fabricated [5]. Direct metal laser sintering (DMLS) is a kind of powder bed fusion in which metal powder is sintered using a powerful laser [6]. Co-Cr, titanium alloys (Ti), Al, and stainless steel (SS) are frequently utilized in DMLS. Thermal conductivity and melting point are two important material qualities to be considered when choosing a material for 3D metal printing [7]. In metal 3D printing, layer thickness (LT) is an essential factor influencing the part strength, build time, and surface finish. Smoother surfaces often result from finer layer resolutions [8], although they can also lengthen construction times [9]. Metal 3D printed objects frequently need post-processing procedures to enhance their mechanical characteristics and surface smoothness. Surface coating, heat treatment, machining, and polishing are standard post-processing methods [10]. Another powder bed fusion method, electron beam melting (EBM), melts metal powder using an electron beam rather than a laser [11]. EBM is frequently used to produce thick [12], totally melted metal powder and is renowned for its rapid build speeds. In directed energy deposition (DED), metal wire or powder is melted using an electron beam or other high-energy heat source [13], and then the melted material is deposited onto a substrate in the desired form [14]. DED is frequently used to add material or repair existing components [14]. The application of 3D metal printing in numerous sectors, including aerospace, automotive, healthcare (e.g., medical implants), and tooling, is widely reported [15]. However, exorbitant prices, constrained construction sizes, and post-processing need special attention. These issues are still being researched, and developments are still being made to improve the capabilities of metal 3D printing technology [16].
Intramedullary (IM) pins are frequently used in veterinary applications to treat canine bone fractures internally [17]. These IM pins stabilize fractures and encourage appropriate healing by being placed into the medullary canal [17], the core chamber of long bones. These materials have been selected based on their strength, ability to withstand corrosion, and suitability for the canine body [18]. The canine’s weight and size, as well as the kind and location of the fracture [19], all affect the IM pin’s diameter and length. The IM pin should be just big enough to prevent further injury to the bone while still having enough diameter to offer stability. IM pins come in various designs, but to improve strength and encourage bone ingrowth, they frequently have a smooth or threaded surface [20]. During surgery, IM pins are implanted after making an incision to reveal the fracture location. The surgeon drills into the medullary canal to place the IM pin [21]. The IM pin can be put down the length of the bone to offer support, or it can be placed across the fracture site to stabilize it. IM pins may occasionally be equipped with locking devices [22], such as bolts or screws, to improve stability and stop the pin from rotating or moving at the fracture site. The canine might need limited activity for a while following the implantation of an IM pin to promote recovery. It is crucial to schedule routine follow-up visits with the veterinarian to track the healing process and manage any issues or difficulties. Canines with bone fractures can often benefit from IM pin stabilization [23]. A veterinary surgeon will usually decide whether or not to use IM pins depending on the specifics of each case and the best course [23] of care for the canine. Materials for IM pins must be biocompatible, mechanically robust, and frequently customized for functions inside the canine body. Various biomaterials are employed for IM pin, each with unique benefits and characteristics [24].
The unique criteria of the implant application, such as load-bearing capability, biocompatibility, and the physiological milieu of the implant site, determine which biomaterial is best [25]. The creation of safer and more functional biomedical implants is facilitated by the ongoing introduction of new biomaterials and the enhancement of current ones through advances in material science. The Ti and its alloys have superior resistance to corrosion, low density, high strength, and biocompatibility. The applications of Ti alloy in dental implants, bone plates, and orthopaedic implants, among others, are well reported [26]. The SS has good strength, resistance to corrosion, and durability and is used in dental implants, vascular stents, and orthopaedic implants [27]. The Co-Cr alloys possess high strength, resistance to corrosion, and biocompatibility. Applications include dental prostheses, cardiovascular stents, and orthopaedic implants [28].
Finite elements analysis (FEA) extensively examines how structures and individual components behave under different circumstances [29]. Using FEA on an IM pin can reveal details about stress distribution, deformation, and overall structural performance. Using SolidWorks software, the IM pin’s geometry was initially modelled. Determining the form, size, and material characteristics is necessary for this step [30]. In the following steps, a mesh was created by breaking the model into separate parts. The mesh’s quality impacts the accuracy of the analysis. The relevant elements in the model assigned the material properties of the IM pin, such as yield strength, poison ratio, and Young’s modulus. The predicted conditions during use serve as the basis for defining loading scenarios, which might involve tensile, compression, and torsional stress or loading for an IM pin [31]. Boundary conditions are specified to replicate the interaction between the pin and the surrounding bone or tissue. The IM pin’s reaction at constant load was analyzed using static analysis as per reported literature [32]. The IM pin’s stress, strain, and deformation under the given circumstances were computed using the FEA.
Orthopaedic implants must undergo corrosion testing to guarantee their long-term stability and biocompatibility with the canine’s body [33]. Saline solutions or their modified forms, such as Hank’s balanced salt solution (HBSS), are often used solutions for corrosion tests (2.5 g of NaCl per 100 mL of water) [34–38]. The corrosion testing was done using the ASTM-G-59 [36–39].
The literature survey was performed on the Web of Science database for the last 20 years with three combinations of keywords: “FEA, Biomaterials, 3D metal printing”. These keywords were inserted in different rows, and 17 results were found. The plain .txt file was further processed with open-source visualization of similarities viewer (VOS) viewer software for the minimum occurrences of term 03. The total number of terms was found to be 744, and 30 terms met the threshold; for each of the 30 terms, a relevance score was calculated, and based on this score, the most relevant terms were selected, and the default choice was to select the 60% most relevant terms as per previous study [40].
Materials and methods
The 17-4-precipitated hardened (PH)-SS is renowned for its strength, corrosion resistance, and simplicity. nickel alloys (Ni)-625 is a high-strength, anti-corrosion Ni-Cr-Mo alloy. Ni-625 has established high-temperature tensile strength and exceptional resilience to various corrosive conditions. Ti-6Al-4V is an alloy commonly utilized in many applications due to its outstanding blend of biocompatibility, strength, and resistance to corrosion. The methodology adopted for this study is shown in Figure 1.
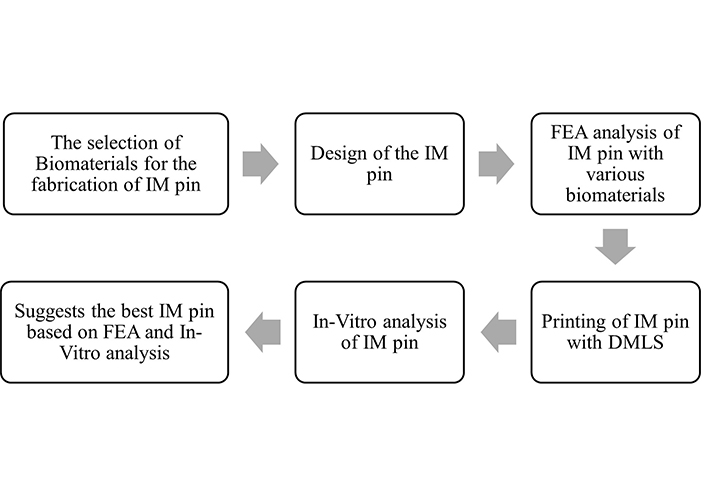
Adopted methodology for the study. IM: intramedullary; FEA: finite elements analysis; DMLS: direct metal laser sintering
The selection of materials is based on the biocompatibility, corrosion resistance, strength, and availability of implant-grade metal powder, i.e., 17-4-PH-SS, Ni-625, and Ti-6Al-4V. After selecting biocompatible metal powder, the following steps were designing the IM pin in SolidWorks software in consultation with a veterinary surgeon.
The FEA for each material (17-4-PH-SS, Ni-625, and Ti-6Al-4V) was performed separately. After FEA analysis, the IM pins were fabricated with 17-4-PH-SS, Ni-625, and Ti-6Al-4V on the DMLS setup. The printing parameters to fabricate the 17-4-PH-SS and Ni-625-based IM pin were: laser power (LP): 120 W, hatch distance (HD): 50 µm, LT: 30µm, scanning speed (Sc.S): 1,200 mm/s, energy density (ED): 66.66 J/mm3, support: wall support inline with literature [10]. The printing parameters to fabricate the Ti-6Al-4V-based IM pin were LP: 190 W, HD: 100 µm, LT: 30 µm, Sc.S: 500 mm/s, ED: 123 J/mm3, support: wall support.
After printing the IM pin, the in-vitro test was performed for each selected material, and the in-vitro test was performed. The final step is ascertaining the best IM pin based on the FEA and in-vitro results.
Results
The simulation study was performed on IM pin through Ansys workbench (version R2-2022, Canonsburg, Pennsylvania, U.S.) software. The deformation, elastic strain, and stress (Von-Mises criteria for failure) of 03 biocompatible materials (17-4PH-SS, Ni-625, and Ti-6Al-4V) under tensile, compressive, and torsion is shown in Table 1. FEA results of biomaterials are based on Von-Mises criteria (applied load of 1,500 N, cell type: tetrahedron, grit size: 0.15 mm, number of nodes: 213,989, and elements: 145,012). The distal end was fixed, and the load was applied to the proximal end (Figure 2).
FEA results of three biocompatible materials
Biomaterials/Test performed | Deformation (mm) | Elastic strain | Stress (σv; MPa) | Yield strength (σy) at 0.2% of strain (MPa) | Safety factor against failure (N) = σy/σv | |
---|---|---|---|---|---|---|
17-4-PH-SS | Tensile | 0.0093946 | 0.00049473 | 93.412 | 625 [23] | 6.69 |
Compression | 0.0093946 | 0.00049473 | 93.412 | 998 [24] | 10.68 | |
Torsion | 0.0088526 | 0.00031084 | 63.331 | 499.86 [25] | 7.89 | |
Ni-625 | Tensile | 0.0085431 | 0.00065708 | 104.12 | 230 [26] | 2.20 |
Compression | 0.0085431 | 0.00065708 | 104.12 | 720 [27] | 6.91 | |
Torsion | 0.0087563 | 0.00030212 | 61.967 | 690 [27] | 11.13 | |
Ti-6Al-4V | Tensile | 0.013869 | 0.0009387 | 98.739 | 1,062 [28] | 10.75 |
Compression | 0.017137 | 0.00093473 | 96.612 | 1,100 [29] | 11.38 | |
Torsion | 0.01728 | 0.00057198 | 61.038 | 970 [30] | 15.89 |
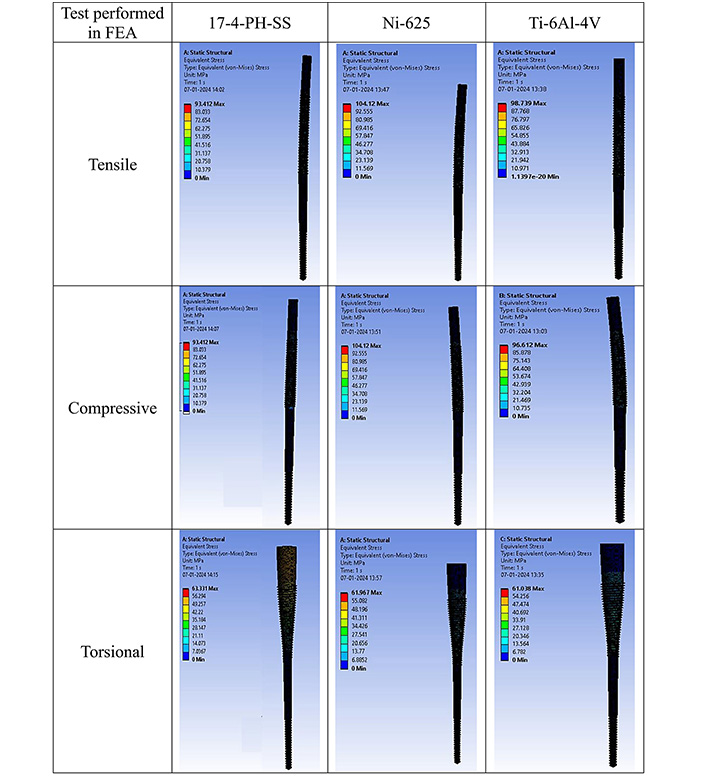
FEA result of equivalent stress in tensile, compressive, and torsional loading. FEA: finite elements analysis
As observed from Table 1, for 17-4-PH-SS, Ni-625, and Ti-6Al-4V the predicted σv values under tensile (93.412 MPa, 104.12 MPa, and 98.739 MPa), compression (93.412 MPa, 104.12 MPa, and 96.612 MPa), and torsional (63.331 MPa, 61.967 MPa, and 61.038 MPa) loading has some variations. As per the literature, the σy at 0.2% of strain for 17-4-PH-SS, Ni-625, and Ti-6Al-4V is also significantly different [23–30]. Finally, based on the maximum “N value” (10.75, 11.38, and 15.89) for tensile, compression, and torsional loading, Ti-6Al-4V has been selected as the best among the 03 options available.
In-vitro analysis of biomaterials-based IM pin
For in-vitro examination, a potentiostat (corrosion testing setup, make: PhadkeSTAT-20, Mumbai, India, with compatible EC-Prayog software) was used. Using a four-electrode unit cell (consisting of a working electrode, a calomel reference electrode, a graphite electrode, and a floating electrode), Figure 3 displays the Tafel plots for biomaterials at 25°C. Utilizing the measured values of open circuit potential (OCP) and linear polarization resistance (Rp), the Tafel plots of three biomaterials are shown in Figure 3. To better recreate the simulated bodily fluid (NaCl of 2.5 g per 100 mL of water), the samples were soaked in the solution (simulated body fluid) for 15 min before the calculation of Tafel [38, 39]. The equations (1) and (2) have been utilized to compute corrosion current (Icorr) and corrosion rate (CR) following the reported literature.
Here, ρ = working material density (in g/mm3), A = exposed cross-sectional area in mm2, EW = equivalent weight in g/mol, and K = constant, βa = anodic constant, βc = cathodic constant, Rp = linear polarization resistance. The observed values of Icorr, CR, βa, and βc of three biomaterials are shown in Table 2.
In-vitro results of biomaterials
Characteristics | Biomaterials | ||
---|---|---|---|
14-4-PH-SS | Ni-625 | Ti-6Al-4V | |
Linear polarization resistance (Rp; Ω) | 26,400 | 162,000 | 479,000 |
Anodic constant (βa) | 0.03451 | 0.10129 | 0.06884 |
Cathodic constant (βc) | 0.17691 | 0.10664 | 0.17692 |
Equivalent weight (EW; g/mol) | 27.92 | 29.34 | 11.96 |
Density (ρ; g/cm3) | 7.8 | 8.44 | 4.45 |
Exposer area (A; cm2) | 1.5 | 1.5 | 1.5 |
Constant (K; mm/year) | 0.00327 | 0.00327 | 0.00327 |
Corrosion current (Icorr; A) | 4.75 × 10–7 | 1.392 × 10–7 | 4.492 × 10–8 |
Corrosion rate (CR; mm/year) | 3.70622 × 10–9 | 1.0552 × 10–9 | 2.63211 × 10–10 |
Table 2 shows the result of the in-vitro analysis of three biomaterials for possibly fabricating IM pins for orthopaedic applications in canines. The minimum CR was found in the case of Ti-6Al-4V, and the maximum CR was observed in the case of 17-4-PH-SS.
Table 3 depicts the surface characteristics of the 03 selected bio-materials based on scanning electron microscopy (SEM) images. Energy dispersive spectroscopy (EDS) analysis was also performed using SEM images. The percentage (%) of porosity representing pores in the IM pin surface was calculated per ASTM B 276 using metallurgical image analysis software. In the context of an IM pin, porosity can affect its strength, durability, and ability to integrate with surrounding tissue. Based on porosity% analysis, Ti-6Al-4V resulted in better control over the porosity post-in-vitro. Grain size No. was calculated as per ASTM E 112, which represents the No. of grains per unit area. Based on grain size No. analysis Ti-6Al-4V resulted in fine grain size, and corresponding better surface roughness (Ra) was observed. For further analysis of surface characteristics, Gwyddion open-source image processing software was used to calculate the amplitude distribution function (ADF), which describes surface features’ amplitude (heights) distribution in terms of the probability of getting the highest peak within a given area. As observed from ADF, the likelihood of getting the highest peak on the surface was observed more in the case of 17-4-PH-SS and minimum in the case of Ti-6Al-4V. The same was counter-verified by the bearing ratio curve (BRC), which is a cumulative probability of getting the highest peak. 3D render image represents the sample’s surface morphology based on SEM images.
Surface characteristics of IM pin after in-vitro analysis
Surface characteristics | Materials | ||
---|---|---|---|
17-4-PH-SS | Ni-625 | Ti-6Al-4V | |
SEM image | ![]() | ![]() | ![]() |
EDS analysis | ![]() | ![]() | ![]() |
%Porosity | ![]() | ![]() | ![]() |
Grain size No. | ![]() | ![]() | ![]() |
Ra | ![]() | ![]() | ![]() |
ADF | ![]() | ![]() | ![]() |
BRC | ![]() | ![]() | ![]() |
3D rendered image | ![]() | ![]() | ![]() |
SEM: scanning electron microscopy; EDS: energy dispersive spectroscopy; ADF: amplitude distribution function; BRC: bearing ratio curve
Discussion
Based on this study, the maximum safety factor against failure (N) [ratio of 0.2% of yield strength (σy) to the Von-Mises stress (σv)] was observed as 10.75, 11.38, and 15.89, respectively, for tensile, compression, and torsional loading in the case of Ti-6Al-4V. Also, the better biocompatible material for the orthopaedic implant application based on the corrosion result is Ti-6Al-4V due to a lower CR (2.63211 × 10–10 mm/year) in comparison to 17-4-PH-SS and Ni-625. Based on CR and surface characteristics, the Ti-6Al-4V is a better material than 17-4-PH-SS and Ni-625 for the intended application. Further studies may be conducted with other bio-compatible materials, focusing on the requirements of stress shielding in metallic implants and destructive testing.
Abbreviations
3D: | three-dimensional |
ADF: | amplitude distribution function |
AM: | additive manufacturing |
BRC: | bearing ratio curve |
CR: | corrosion rate |
DED: | directed energy deposition |
DMLS: | direct metal laser sintering |
EBM: | electron beam melting |
EDS: | energy dispersive spectroscopy |
FEA: | finite elements analysis |
HBSS: | Hank’s balanced salt solution |
Icorr: | corrosion current |
IM: | intramedullary |
LT: | layer thickness |
Ni: | nickel alloys |
OCP: | open circuit potential |
PH: | precipitated hardened |
Rp: | linear polarization resistance |
SEM: | scanning electron microscopy |
SS: | stainless steel |
Ti: | titanium alloys |
βa: | anodic constant |
βc: | cathodic constant |
Declarations
Acknowledgments
The authors acknowledge the research support provided by the National Institute of Technical Teachers Training and Research Chandigarh and Prof. Ashwani Kumar from Guru Angad Dev Veterinary and Animal Sciences University Ludhiana.
Author contributions
MH: Investigation. RS: Conceptualization. BSP: Supervision. GS: Investigation. JPD: Project administration.
Conflicts of interest
The authors declare that they have no conflicts of interest.
Ethical approval
Not applicable.
Consent to participate
Not applicable.
Consent to publication
Not applicable.
Availability of data and materials
The authors declare that the data may be available on request.
Funding
The authors was supported by the Department of Science and Technology for funding under FIST Level-0 [Project No. SR/FST/College-/2020/997]. The funders had no role in study design, data collection and analysis, decision to publish, or preparation of the manuscript.
Copyright
© The Author(s) 2024.