Abstract
The aim of this contribution is to analyze and discuss the perturbations of body-onboard medical devices caused by electromagnetic field radiations. This involves their control via electromagnetic compatibility analysis and their protection against such perturbations. The wearable, detachable, and embedded devices are first presented and their monitoring, control, forecasting, and stimulating functions are detailed. The interaction of these devices with field exposures comprising their wireless routines is then analyzed. The perturbations control of onboard devices is investigated through the mathematical solution of governing electromagnetic field equations and their appropriate protection strategies are deliberated. The involved investigations and analyses in the contribution are supported by a literature review.
Keywords
Body-onboard devices, electromagnetic fields, electromagnetic compatibility analysis, functional perturbations, shieldingIntroduction
Throughout the historical existence of humanity, the target of modernism has never ceased. In our time, many daily procedures and devices characterize contemporary human society. The connection of these assistive accessories with humans leads, in addition to the desired objectives, to undesirable effects. These adverse effects can disrupt not only humans but also wildlife (One Health concept) and other entities. This is due to the proximity of the environment of humans to that of these others. The operation of one of the most popular categories of such equipment is concomitant with electromagnetic fields (EMFs), particularly wireless ones. Typical examples of wireless communication devices are telephone cells and their tower antennas, and wireless power transfer, such as charging and heating devices.
It is certain that energy transfer and wireless communication technologies contribute greatly to the well-being of society. The presence of devices using such technologies in daily use is constantly increasing. Due to their operating principle, in addition to their functional transmission of waves, they emit parasitic (stray) EMFs. These EMF exposures can interact with objects and living tissues in general [1–15]. Additionally, these wireless devices can interact with each other and each could disrupt their operation. Thus, consolidating the desired objectives of these tools and reducing their harmful side effects is the subject of an emerging challenge [16].
The growth of body-onboard medical devices has placed emphasis on user well-being, acceptability, security, and improved medical operability. Different categories of sensors have been transformed into wearable, detachable, and implanted configurations to further monitor body indications and enable instantaneous and uninterrupted detection. To achieve these body-sensing records, it is essential to incorporate wireless, power delivery, and information communication routines alongside embedded devices.
It is apparent from the above discussion that body-onboard devices in general, coupled with their wireless power transfer and data communication could interact with other medical or everyday use wireless devices mentioned above. The latter can act as a source of EMF radiation disrupting the detections of the exposed body-onboard devices and their wireless connections. Various other adverse side effects of EMF radiation, in addition to detection functions, could perturb medical devices. Typical examples of potential side effects of EMF are premature drug release of delivery tools and heating of metallic implants.
The purpose of this paper is to investigate and highlight the effects of the EMF interaction with portable, connectable, and embedded devices, as well as their protection strategy against such effects.
Three sections constitute the present work, the details and functions of body-onboard devices, their interaction with EMFs, and the governing equations permitting control analysis and protection. First, portable, detachable, and embedded devices will be presented and their monitoring, control, prediction, and stimulation functions will be described. In the second part, the interaction of these devices with EMF exposures including their wireless routines will be analyzed. The third section concerns the mathematical equations governing EMFs and their numerical solution in the context of device functionality control. Thus, an electromagnetic compatibility (EMC) analysis is carried out using such a solution to verify their integrity (constancy). At the end of this section, shielding strategies will be discussed and analyzed. The involved investigations and analyses in the contribution are supported by a literature review.
Wearable, detachable, and embedded devices
Three categories of body-onboard devices are usually employed, which include wearable, connectable (or detachable), and implanted (or ingestible) that can be passive or active. These devices possess generally smart behavior, i.e., they reflect a facility to extract data in a smart manner and in real-time. This section is dedicated to the analysis of their roles in healthcare.
Monitoring, control, forecasting, and stimulating functions
Non-invasive wearable devices that operate in real-time enable uninterrupted monitoring of the people being cared for and thus provide appropriate health information to determine their overall condition of health and, moreover, a primary picture of health assessment [17–22]. Additionally, connectable or detachable intelligent detecting tools that besides serve as instantaneous condition supervising means provide personally useful warnings related to physical disorders. These warnings relate to heart rate, pressure of blood circulation, rate of respiration, etc. This personalized healthcare monitoring delivers relevant medical data [23–25]. Moreover, modern advances in biocompatibility and biodegradability of materials [26–37] have facilitated advancements in the field of integrated fixed inactive tools enabling identification and prediction by means of miniature sensors, thus intensely improving the value and the effectiveness of individual health care [38–42]. Other embedded devices, stationary but active, are intended to stimulate or activate a part of the body, such as cardiac pacemakers, neurostimulators, or pumps [43–47].
Post-treatment and connected personalized home clinics
In addition to the mentioned roles of monitoring, forecasting, stimulation, etc. of the mentioned devices, these also make it possible to supervise the post-treatment condition of patients [48, 49]. Moreover, following health management recommendations, the paradigm of personalized healthcare through a connected integrated strategy is booming. Thus, replacing face-to-face care with connected assistance using the three mentioned categories of body-onboard tools in individualized care [50–54].
Wireless power and communication routines
Wireless, power supply, and information communication routines are necessary for the intelligent operation of body-onboard tools [55]. This important feature of an onboard device reflects some vulnerabilities. Besides the conventional security of transmitting personal data via wireless communication networks [56], the physical nature of wireless could experience EMF exposures. Thus, in addition to the harmful effects of external exposure to EMFs on the body-onboard tool itself, this can disrupt its wireless transmission procedures.
Interaction of onboard devices with EMF
A notable security issue concerns the protection of the above onboard devices against EMF noise radiated by neighboring everyday equipment. In fact, wearable devices typically incorporate electronic and optical biosensors that provide real-time data on a patient’s electrophysiological or biochemical status [57]. These sensors could be affected by EMF radiation; thus, their functions of detecting the patient’s condition would be erroneous. A specific example could be a wearable drug delivery device with silicon nanomembrane strain sensors, a temperature sensor, and electroresistive heaters [19]. In this example, exposure to EMFs could affect the operation of the device. This can be undertaken using various approaches. Firstly, avoiding EMF-sensitive ingredients in their constitution as much as feasible. Another possibility is to defend, by protecting such onboard tools along with the radiation sources. Another safety measure is to keep these onboard devices away from tough EMFs, for instance being inserted into the strong MRI magnet. The safety of these devices, in addition to their protection, can be controlled by EMC routines.
EMC onboard devices control
A significant attribute of the position of such a tool (recipient) versus radiation (supply) is if it were able to function as usual in a radiation environment, this defines its performing aptitude. Nevertheless, once this receiving tool undergoes field radiation, its key performance signs might be disrupted by a reduction in the signal-to-noise ratio, signal carryover, etc., and therefore, its operation might be reduced. Subject to the domain of use, a receiving tool has certain practical requests and its EMC control assessment relates to subsequent criteria [58, 59]. Such criteria are principally established on static methods. So, the EMC check examination achieved on the tool is built on a universally mounted waveform whereas the control is executed based on the results of the test. This convolution of reflecting a complex EMF radiation environment dynamic behavior is challenged by the device function. Several researches have been achieved to enhance the verification methods of EMC evaluation [60–66].
The experimental control techniques designated overhead are rather complicated and frequently need specialized and costly protected locations. In such circumstances, an alternate answer can be verified by numerical computations through an EMC study to confirm the reliability of different concerned devices [67–70]. Indeed, the legitimacy of an approach guarding a tool against field radiation might be ensured by an EMC examination verifying the invariance of device fields due to radiation.
Governing equations, EMC analysis, and shielding
This part regards the EMC check mentioned above relating to onboard medical devices. Such a control is established and ruled by the equations of EMF characterizing the field’s comportment. This verifies the possible disturbance of local distributions of induced EMFs in an EMF-sensitive object due to a given field radiation. Note that, if the radiated target were insensitive to EMF, its field distributions would not be changed.
EMFs governing equations
The general EMF four equations, in their differential form, based on Maxwell’s microscopic local equations [71] are given by:
∇ × E = − ∂t B (Maxwell – Faraday), ∇ × H = σ E + ∂t D (Maxwell – Ampère), ∇ · D = ρe (Maxwell – Gauss), and ∇ · B = 0 (Maxwell – Thomson).
For harmonic fields’ case, the EMF equations can be given by:
(1) ∇ × H = J
(2) J = Je + σ E + j ω D
(3) E = −∇ V – j ω A
(4) B = ∇ × A
In the above EMF equations, H and E are the vectors of the magnetic and electric fields in A/m and V/m, B and D are the vectors of the magnetic and electric inductions in T and C/m2, A and V are the magnetic vector and electric scalar potentials in W/m and volt. J and Je are the vectors of the total and source current densities in A/m2, σ is the electric conductivity in S/m, ρe is the volume density of electric charges in C/m3, and ω = 2πf, where f is the frequency in Hz of the exciting EMF. The vector ∇ is a partial derivative operator, with three likely inferences gradient (product with a scalar field), divergence and curl (dot and cross products respectively, with a vector field). The sign ∂t corresponds to the operator of partial time derivative. The magnetic and electric behavior laws respectively between B/H and D/E correspond to the permeability μ and the permittivity ε in H/m and F/m.
The excitation in (1–4) is current density Je = σ Ee = j ω De = j ω ε Ee. The choice of the form of the source term depends on the nature of the exposure and the exposed material nature.
Equation solution and EMC
In general, depending on the geometric complexity and inhomogeneity of the materials, the solution of (1–4) must be local in the device using 3D discretized methods as finite elements [72–79] in the appropriate element of the material. The discretized 3D elements are volume sections delimited by surface elements, each bordered by edge elements, each terminated by two nodes. Fields could be expressed at the level of nodes, edges, faces, or volume depending on the nature of the field such as field continuity constraints, etc.
An EMC analysis aims to monitor, through the solution of EMF equations, disturbances due to an external source of EMF exposure on a recipient tool, revealing its measure of EMF reaction. In the present work, we need to verify the onboard devices’ inattention to EMF exposures. Such control may be accomplished by matching the distributions of fields in the device with and free of interfering sources. The field constancy in shielded onboard devices will be checked without and with exposure. Actually, for a certain source field Ee linked to Je, solving (1–4) will provide the induced values of the EMF, Ei, Bi, and Ji in each element of the discretized domain. The resulting distributions of EMFs allow the control of EMC. The solution domain in this case corresponds to the structure of the device. The verified EMC control due to an EMF exposure involves the constancy confirmation of the field values in the domain. Such an EMC check could be accomplished on the single being checked instrument to ensure its own running. Still, such devices generally operate neighboring to living tissues and can act together. Such a coupling can modify the comportments of the device along with the tissues [80].
Smart shielding
As stated previously, to decrease the influences of radiation of EMF on onboard medical tools, we can manage each shielding of the recipient and radiation supply or modify each of the constructions employing tools for design and optimization. These approaches can be supported and confirmed by control through EMC analysis. Protecting equipment by shields in general employs constituents displaying behaviors of absorbing or reflecting the radiated fields to inhibit its traversing between the two borders of the shield. Electromagnetic interference (EMI), coverings, sheets and shields, which support holding EMF radiation, are necessary for the customary action of medical tools and the protection of living tissues. Magnetic and electric fields composing an EMF wave are directed in the space perpendicularly. Thus, EMI shielding methods are categorized into EMI electric, magnetic, or their coupling shields. It should be noted that high-frequency EMF waves, such as RF waves, mainly distinguish radiation, showing interrelated electric and magnetic fields. So, if one of the two fields is shielded, the other will also be hidden. Due to this occurrence, shields are taken simply as electric conductives. However, shields with a simple conductive material could exhibit, under EMF exposure, induced currents, which would involve EMF dissipated losses causing heating. The closeness of the shielded device to tissues could cause temperature rise and adverse effects on body tissues [80]. In such conditions, the conductive nature of the shield should be adapted to account for this body tissue adverse effect. In general, the function of target nature and shielding technologies use materials of different types. These include clothing fabrics, adhesives rubbers, and coatings. Such diverse constituents are associated with the needed elasticity, the fixative ability, the easiness of processing, and consistency. The investigation domain of protective shields is very dedicated because of the development of everyday EMF exposures [81–92]. Concerning the adjustment of the shield conductive character indicated above, the use of multifunctional accorded substances for shielding low-reflectivity could present improved defense. The matter adapting can decrease the tough EMF reflection triggered by the matter’s high conductivity. Furthermore, a particular fabricating procedure allows diminishing of the substance coefficient of reflected power, along with lessened heat dissipation and enhanced isolation and kind ecologically defending substances [93–95]. In ending, solitary a tool built of EMF-indifferent constituents or smart shielded, can be safe.
Additional remarks
Following the analyses reported in the last sections, which were limited to the situation considering that living tissues do not affect and are not affected by the occurrence of exposure to EMF. In fact, onboard devices operate in close proximity to living tissues and can jointly interact with EMFs. Thus, the behavior of devices due to exposure to EMF would be influenced by the presence of living tissue. Moreover, in addition to likely thermal biological effects, living tissues could be heated due to the increased temperature of metal parts of devices or their shields due to EMF exposure. Accordingly, EMF control could include the onboard device associated with the phantom of a part of living tissue modeled by EMF coupled with bioheat equations. Figure 1 illustrates a summary diagram of onboard device control strategies and EMF exposure behaviors in the generally mentioned case.
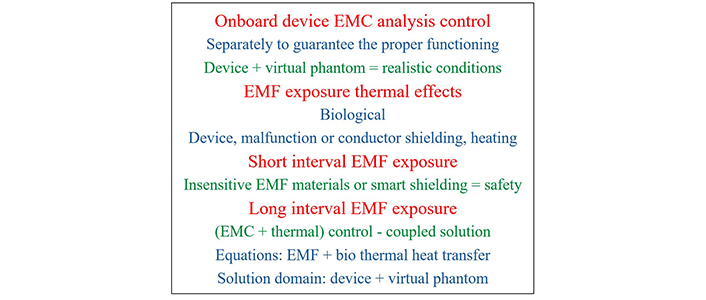
Summarizing diagram of control strategies of onboard devices and EMF exposure accounting to living tissues and thermal effects
Besides the mentioned side effects of EMF on living tissues and associated with embedded devices, an important effect could be encountered regarding the generation of reactive oxygen species, which can particularly affect the healing of inflammatory tissues. In fact, onboard devices often use Bluetooth, which operates in the microwave range and would be responsible for such effect [96].
Conclusions
In this contribution, we analyzed and discussed the disturbances due to EMFs on body-onboard medical devices. The control via EMC analysis of device disturbances was studied through the mathematical solution of the equations governing the EMFs. The research and analysis involved in the contribution was supported by a literature review. Several concluding remarks are worth mentioning:
Numerical EMC analysis could be an effective tool to verify the integrity of the devices studied. Protection of these devices could be ensured by avoiding the presence of unprotected sources of exposure, using smart shields on devices, and developing radiation presence detectors on devices.
Abbreviations
EMC: | electromagnetic compatibility |
EMFs: | electromagnetic fields |
EMI: | electromagnetic interference |
Declarations
Author contributions
AR: Conceptualization, Data curation, Formal analysis, Writing—original draft, Writing—review & editing. The author read and approved the submitted version.
Conflicts of interest
The author declares that he has no conflicts of interest.
Ethical approval
Not applicable.
Consent to participate
Not applicable.
Consent to publication
Not applicable.
Availability of data and materials
Not applicable.
Funding
Not applicable.
Copyright
© The Author(s) 2024.