Abstract
Surgery, chemotherapy, radiation therapy, and immunotherapy are potential therapeutic choices for many malignant and metastatic cancers. Despite adverse side effects and pain, surgery and chemotherapy continue to be the most common cancer treatments. However, patients treated with immunotherapy had better cancer control than those who got other treatments. There are two methods to activate immunological pathways: systemically and locally. To modify the tumor microenvironment (TME), the former uses systemic cytokine/chemokine (CK) delivery, whilst the latter uses immunological checkpoints or small molecule inhibitors. Organic and inorganic nanomaterials (NMs) enhanced the efficacy of cancer immunotherapy. NMs can transmit drugs, peptides, antigens, antibodies, whole cell membranes, etc. Surface-modified NMs precisely target and enter the tissues. The inner core of surface-modified NMs is composed of chemicals with limited bioavailability and biocompatibility, resulting in prolonged blood retention and decreased renal clearance. These platforms hinder or prevent many immune cell activities and modify the TME, enhancing the efficiency of cancer immunotherapy. By inhibiting CK/CK receptor signaling, cell migration and other immune responses could be controlled. Developing CK-targeted nanoparticles (NPs) that inhibit CK signaling or take advantage of the ligand-receptor connection is possible. Surface chemical modification of NMs with CKs or specific peptides has several medicinal applications, including tissue-specific drug delivery and limited cell migration in cancer-afflicted conditions. This review covers current developments in the role of different groups of CK-loaded NP in tumor therapy targeting immune cells and cancer. It also covers the role of NP targeting CK signaling which aids in immunogenic cell death (ICD) and induction of antitumor immunity. In addition, CK gene silencing and its capacity to prevent cancer metastasis as well as inhibition of immune cell migration to modulate the TME are discussed.
Keywords
Chemokine, immunogenic cell death, chemokine receptors, nanomaterials, cancer, therapeutic relevanceIntroduction
The tumor microenvironment (TME) is a heterogeneous ecological niche, characterized by an exceptionally diverse population of both tumor and infiltrated immune cells [exhausted lymphocytes and immunosuppressive population like regulatory T (Treg) cells] and fibroblast cells [1]. The growth of cancer and the spread of cancer to other parts of the body are both facilitated by chemokine (CK) and CK receptors. A wide variety of CK receptors have been explored. CKs are released by host cells into surrounding tissue in a concentration gradient manner that influences the leukocyte movement [2]. Their conventional function is to mediate the migration of many different immune cell types throughout the body and also to the TME [3]. Nanocarriers might be utilized to improve the immunogenicity of cancer cells that are dying, reducing the adverse effects of chemotherapy and the difficulties associated with drug resistance. Notably, the impact of nanomaterials (NMs) on the human immune system is a primary issue when assessing the health effects of such low-dose, long-term, repeated exposure conditions. Due to their exceptional physical, chemical, and biological properties, nanoparticles (NPs) have played significant roles in the biology of clinical medicine [4]. NMs offer several potential applications the in detection, imaging, and therapy of cancer due to their small size and amenability to modification [5]. In order to decrease therapeutic dose while avoiding the associated unwanted effects, nanocarriers for drug delivery have been a sought-after strategy with the highest possible precision. The fundamental strategy includes changing the chemistry of the NP surface to provide the exact targeting of receptors, expressed exclusively or mostly in the specific cell type. Immune cells that have undergone differentiation express diverse CK receptor patterns, and the same cell may upregulate or downregulate these receptors at various stages of its life cycle [6]. The use of organic and inorganic NMs has improved cancer immunotherapy effectiveness (Figure 1). The NP sizes range from 1 nm to 200 nm with distinct physiochemical characteristics. Many research organizations have proved the effectiveness of NMs in cancer detection and therapy [7, 8]. All of these ideal properties are advantageous in treating widely dispersed malignancies with minimum damage to the system [9, 10]. The peptide [11], antigen [12], antibody [13], whole-cell membrane [14], drugs [15], aptamer [16], and also the active targeting [15, 17] are all successfully delivered by NMs. These compounds specifically inhibit or block multiple immune cell checkpoints.
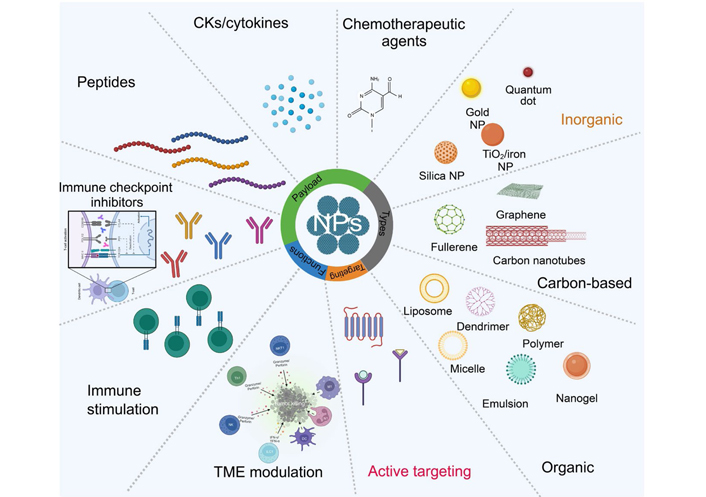
An overview of NMs and their potential use in cancer immunotherapy. NPs made from metal ions, biodegradable polymers, lipids, and cell membranes have all been implicated in cancer immunotherapy. NMs are employed as carriers of immune system activation or inhibition. Peptides, antigens, antibodies, and chemotherapeutic agents are integrated into the inner core or surface of the NMs. NKT1: natural killer T 1; Th1: T helper 1; ILC1: innate lymphoid cells; TNF-α: tumor necrosis factor-alpha; IFN-γ: interferon-gamma; →: apoptosis of cancer cell by perforin and granzyme
This review covers recent developments in the role of various CK-loaded NPs in tumor therapy targeting immune and cancer cells. Additionally, it covers the role of NPs targeting CK signaling, which helps in the immunogenic death of the cancer cells and induction of antitumor immunity. Finally, it covers CK gene silencing and its ability to prevent cancer metastasis and the migration of other immune cells to modulate the TME.
Immunogenic cell death and induction of antitumor immunity
Immunogenic cell death (ICD), characterized by the release of tumor-associated antigens (TAAs) and tumor-specific antigens (TSAs) like neoantigens, damage-associated molecular patterns (DAMPs), and pro-inflammatory cytokines facilitates the presentation of TAAs and TSAs to adaptive immune cells, eliciting an anti-cancer immune response. In cancer, ICD related to DAMPs, including the expression of membrane-anchored calreticulin (CRT) and the heat shock proteins 70 and 90, correlates with the recruitment and activation of anti-cancer immune cells and is the positive prognostic indicator for cancer patients [18]. Ideal cancer treatment activates systemic immunity while killing of cancer cells may be aided by activating ICD in tumors. NPs can transport antibodies and ICD-inducing drugs to tumors, altering the TME and enhancing immune responses [19]. NPs could modify the pharmacokinetics and biodistribution of ICD-inducing drugs, which might aid cancer immunotherapy. Reportedly, chemotherapeutic drugs that cause ICD include anthracycline, taxane, oxaliplatin, cyclophosphamide, bleomycin, and bortezomib [20, 21]. Liposomes, inorganic or polymer NPs, and tumor-derived NPs may deliver immunogenic chemotherapeutic medicines. NPs may be developed to combine immunogenic chemotherapeutic drugs with anti-programmed cell death 1 (anti-PD-1), anti-PD-ligand 1 (anti-PD-L1), or anti-cluster of differentiation 47 (anti-CD47) antibodies to promote ICD antitumor immunity [22, 23]. NP-mediated cancer immunotherapy research might explore how NPs release their payload in the TME. The mesoporous silica NP (MSNP) decorated a triple stimuli-responsive drug delivery platform for the delivery of doxorubicin (Dox) and 5-fluoro-2-deoxyuridine (5FdUrd), which included folic acid (FA) as a ligand to target cancer cells. Matrix metalloprotease 2 responsive liposomes folate-poly(ethylene glycol) (PEG)-liposomes (PEGFALip), which are made of cleavable PEG chains covering folate modified liposome, are being made to deliver Dox, which causes ICD [24, 25]. In recent years, “all in one” multifunctional NPs have been developed to boost cancer immunotherapy efficiency by triggering tumor cell ICD, attracting immune cells, and modulating inhibitory TME. Yang et al. [26] created a pH-responsive copolymer PEG block cationic polypeptide (PEG-b-cPP) nanovesicle to encapsulate photosensitizer and indoleamine 2,3-dioxygenase (IDO) inhibitor indoximod (IND) through hydrophobic contact. After laser irradiation, this smart NP destroyed cancer cells through reactive oxygen species (ROS) and attracted dendritic cells (DCs) for immune activation. The disclosed IND also reversed immunosuppressive TME [26]. This smart NP shows the possibility of the rational NP design for cancer immunotherapy. In addition to manufactured NPs, certain cancer cells release anti-cancer NPs. Wan et al. [27] found that irradiated tumor cells derived microparticle triggered ICD through ferroptosis. In this study, RT-MP cell release after intrapleural injection, and polarized M2-tumor-associated macrophage (TAM) to M1-TAM in a malignant pleural effusion (MPE) animal model, ablating MPE [27].
In experimental cancer therapeutics, few anti-cancer agents ICD and even fewer are therapeutically accessible. To circumvent this, NMs may be employed to boost the immunogenicity of cancer cells, destroyed by anti-cancer therapy, which isn’t immunogenic by themselves. In the last decade, it’s become evident that anti-cancer therapy gets more effective when medication distribution, active targeting, and ICD were employed concurrently. Apoptosis and necroptosis are covered by ICD. Anti-tumor immune responses are triggered by DAMPs in association with cytokines/CKs. The NPs can modify cancer cell killing and immunogenicity. Immunotherapy builds anti-tumor immunity in the immune system to combat cancer. Combining chemotherapy, radiation, and immunotherapy to increase anti-tumor immunity eradicates tumors, and creates long-term immunity. Vaccination using ICD is likely to be the new immunotherapy method [28]. The NPs loaded with chemotherapeutics could induce ICD and can also control immunogenicity (Figure 2) [29]. ICD is a catch-all name for a variety of methods of controlled cell death. DAMPs and cytokines/CKs are the major mediators of the immunogenic features of ICD [30, 31]. These molecules perform critical biological functions inside the cells and aren’t recognized by the immune system. ICD involves the release of DAMPs from dying tumor cells. These DAMPs cause tumor-specific immune responses to be activated, which increases the long-term effectiveness of anticancer drugs by killing cancer cells directly and boosting the immune system’s ability to fight cancer. The ICD hypothesis has recently grabbed the expert’s interest as a cancer immunotherapy strategy. Using a range of NMs may help to solve significant challenges and requirements in ICD control and changing cancer cell-killing strategies. Both cytokines and CKs attract antigen-presenting cells (e.g., DCs), which engulf dying cancer cells [32]. Following the first morphological description of apoptosis, it was widely assumed that tumor cells undergoing apoptosis steer the extracellular environment to an anti-inflammatory state and thus contribute to an immunosuppressive network at the primary tumor site, promoting further tumorigenesis [33]. In anti-cancer therapies, such as chemotherapeutics (e.g., Dox and oxaliplatin) [33], gamma-irradiation [34] and photodynamic therapy [35] trigger a kind of apoptosis known as immunogenic apoptosis. This type of cell death is connected with the production of DAMPs [for example, surface exposure of CRT, adenosine triphosphate (ATP) secretion, and high mobility group box 1 (HMGB-1) release]. DAMPs operate as adjuvants, activating membrane-bound or cytoplasmic pattern recognition receptors (PRRs), [such as Toll-like receptor 4 (TLR4)], phagocytic or scavenger receptors [e.g., low-density lipoprotein receptor-related protein/CD91], and purinergic receptors (e.g., P2RX7/P2RY2). DAMPs induce DC maturation, promoting the generation of anticancer immune responses. It has been demonstrated that delivering ICD inducers packed into NPs considerably boosts their ICD potential [36].
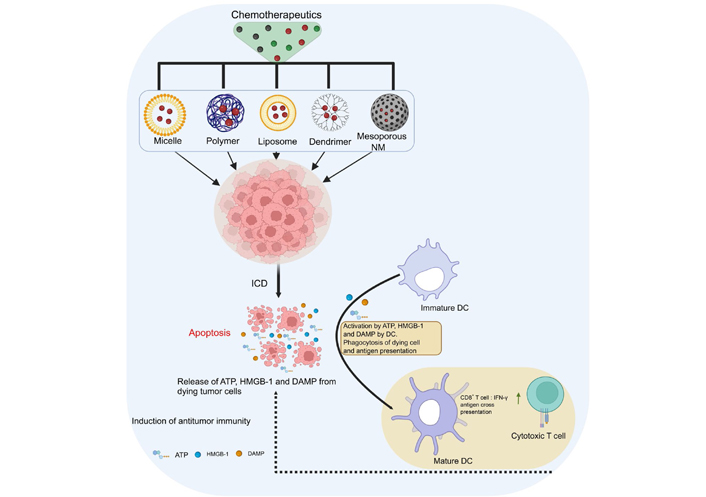
Innovative strategies for regulating the immunogenicity of ICD. In cancer research, NMs are often used to enhance the performance of chemotherapeutics. Immunogenicity and anticancer activities are both enhanced by the delivery of chemotherapeutics in the form of NMs (such as polymer NPs, micelles, dendrimers, mesoporous silica, and liposomes). →: various loaded NMs with drugs targeting the tumor mass; : increases the IFN-γ secretions; ∙∙∙∙∙→: cytotoxic T cell kills the cancer cells
ICD is crucial for cancer immunotherapy using NPs. Further cell death forms, including anoikis, autophagy, pyroptosis, ferroptosis, necroptosis, parthanatos, and NETosis, have been found in recent decades, and more efforts are required to uncover ICD drugs or modalities. An ideal ICD inducer should be able to stimulate the release of a wide variety of DAMPs (such as HMGB-1, CRT, ATP, and other similar molecules), TLR agonists (mitochondrial DNA, ribonucleoproteins, and histones), and immunogenic signals [such as interleukin-1 (IL-1), IL-33].
CK receptor/CK ligands and cancer
CKs, also known as chemotactic cytokines, are small chemoattractant molecules that are released and regulate cell positioning and recruitment into tissues. They are important in embryogenesis, tissue development, and immune response. CKs and their receptors are crucial mediators of leukocyte and cancer cell movement [37]. So far, over fifty CKs and twenty CK receptors have been identified, and in addition to their well-known activities in migration and inflammation of the immune cells, they also play an important role in tumor initiation, development, and progression [38, 39]. CK signaling has been implicated extensively in the regulation of tumor cell decisions toward metastasis [40]. Indeed, the discovery of a novel CK receptor array on cancer cells lends credence to the idea that CKs might specify the secondary destination of propagating tumor cells. CKs are broadly classified into two major classes based on their primary functions: inflammatory CKs and homeostatic CKs. C-X-C motif CK ligand 1 (CXCL1), CXCL2, CXCL3, CXCL5, CXCL7, CXCL8, CXCL9, CXCL10, CXCL11, and CXCL14 are examples of inflammatory CKs secreted during inflammation. Homeostatic CKs such as C-C motif CK ligand 14 (CCL14), CCL19, CCL20, CCL21, CCL25, CCL27, CXCL12, and CXCL13, are constitutively produced and play pivotal roles in homeostatic leukocyte trafficking. CK receptors comprise of 7-transmembrane domain (G protein-coupled), are further classified into subfamilies depending on the pattern of cysteine residues: CXC, CC, and CX3C, where C symbolizes cysteine and X non-cysteine amino acids (Table 1) [41].
CK receptors, ligands, and their role in cancer
CK receptors | Ligands | Role | Cancer type | Immune cells involved | References |
---|---|---|---|---|---|
C-C motif CK receptor 1 (CCR1) | CCL3, CCL4, CCL5, CCL7, CCL8, CCL13, CCL14, CCL15, CCL16, CCL23 | Innate immunity and adaptive immunity | Breast cancer, lung cancer, prostate cancer, cervical cancer, hepatocellular carcinoma, multiple myeloma, T cell leukemia, osteosarcoma, colorectal cancer | Monocytes, macrophages, neutrophils, T helper 1 (Th1) cells, basophils, and DCs | [42] |
CCR2 | CCL2, CCL7, CCL8, CCL12, CCL13 | It helps monocytes move toward chemotactic signals and can be found on the surface of monocytes, basophils, activated memory T cells, and B cells | Breast cancer, pancreatic ductal, adenocarcinoma, glioma, lung cancer, prostate cancer, melanoma, multiple myeloma | TAMs, myeloid-derived suppressor cells (MDSCs), monocytes | [43, 44] |
CCR3 | CCL5, CCL7, CCL11, CCL13, CCL15, CCL24, CCL26, CCL28 | Expressed in several tissues’ keratinocytes, mast cells, Th2 cells, CD34+ hematopoietic progenitors, eosinophils, and basophils | Breast cancer, cervical cancer, renal cancer | Platelets | [45] |
CCR4 | CCR2, CCL3, CCL5, CCL7, CCL22 | CCR4 is preferentially expressed in Treg and Th2 cells. The expression can be transiently upregulated following T-cell receptor (TCR) and CD28 engagement | T cell leukemia, Hodgkin lymphoma, breast cancer, melanoma, hepatocellular carcinoma | Treg cells, monocytes, platelets | [46] |
CCR5 | CCL3, CCL4, CCL5, CCL8 | It is the major co-receptor associated with vulnerability to human immunodeficiency virus type 1 (HIV-1) infection. It is expressed by several cell types, including peripheral blood-derived DCs, CD34+ hematopoietic progenitor cells, and memory T cells | Breast cancer, cervical cancer, lung cancer, multiple myeloma, osteosarcoma, pancreatic cancer, prostate cancer | TAMs | [47, 48] |
CCR7 | CCL19, CCL21 | Expressed in lymphoid tissues and in B and T cells that have been activated. Controls the movement of memory T cells to irritated tissues and promotes the maturation of DCs | Breast cancer, gastric cancer, colorectal cancer, lung cancer, esophageal cancer, B cell leukemia | T cells, Th22 cells, T cells, Treg cells, DCs | [49] |
CCR8 | CCL1, CCL4, CCL17 | Immune surveillance in the skin, type 2 adaptive immunity, and thymopoiesis | Breast cancer | Th2 cells, Treg cells, skin tissue resident memory T (Trm) cells, γδ T cells, monocytes, and macrophages | [50] |
CCR10 | CCL27, CCL28 | Most often expressed by Th2 lymphocytes in the thymus | Leukemia, melanoma | Treg cells | [51] |
C-X-C motif CK receptor 3 (CXCR3) | CXCL9, CXCL10, CXCL11 | Mostly seen on T lymphocytes. It is quickly turned on in naive cells after they are activated, and it stays high in Th1 CD4+ T cells and effector CD8+ T cells | Pancreatic cancer, prostate cancer, breast cancer | Th1 cells, CD8+ central memory T (Tcm) and effector memory T (Tem) cells, natural killer (NK) cells, NKT cells, plasmacytoid DCs (pDCs), B cells. Treg cells, and T follicular helper (Tfh) cells | [52] |
CXCR4 | CXCL12 | Hematopoiesis, organogenesis, and bone marrow homing | Breast cancer, prostate cancer, gastric cancer, esophageal cancer, endothelial cells, ovarian cancer | MDSCs, TAMs, neutrophils | [53] |
CXCR5 | CXC13 | B cell and T cell trafficking in lymphoid tissue to the B cell zone or follicles | Lymphomas, pancreatic cancer, colon cancer, head and neck carcinomas | B cells, Tfh cells, T follicular regulatory (Tfr) cells, and CD8+ Tem cells | [54] |
CXCR6 | CXCL16 | Preferentially expressed on Th1 T cells. CXCR6 has been identified as a minor co-receptor for HIV-1 infection and in adaptive immunity | Breast cancer cells, gastric cancer cells, hepatocellular carcinoma cells, non-small cell lung cancer cells | Th1 cells, Th17 cells, γδ T cells, ILCs, NKT cells, NK cells, plasma cells, and endothelial cells | [55] |
C-X3-C motif CK receptor 1 (CX3CR1) | C-X3-C motif CK ligand 1 (CX3CL1) | Patrolling monocytes in innate immunity, microglial cell and NK cell migration, and type 1 adaptive immunity | Pancreatic cancer, prostate cancer, breast cancer | TAMs, resident monocytes, macrophages, Th1 cells, CD8+ Tem cells, NK cells | [56, 57] |
Importantly, certain CKs can bind to and communicate through many CK receptors, both canonically and atypically [58]. Inappropriate expression and regulation of CK/CK receptors have been related to numerous disorders, particularly those associated with inflammation, such as cancer, where CK receptor expression typically correlates with prognosis [59]. Immune cells, including TAMs and tumor cells, express CK receptors preferentially [60]. Cancer entities including breast cancer, ovarian cancer, cervical cancer, prostate cancer, and glioma have been shown to express numerous CKs and their receptors (Figures 3 and 4) [61].
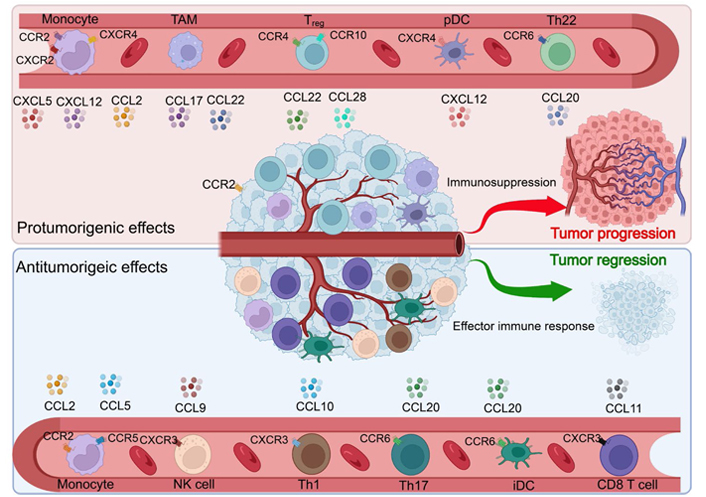
Activation and deactivation of the immune system by various CKs in a tumor setting. Various hallmarks of cancer have been linked to the actions of CKs, CK receptors, and CK-receptor combinations. iDC: interstitial DC
Role of gene silencing against CK receptors and ligands in combination with NM for anti-neoplastic therapy
CK receptors mediate cell chemotaxis in a gradient fashion, demonstrating episodes of attraction events for the immune cells. CK receptors are categorized based on conserved cysteine residues (C, CC, CXC, and CX3C). In malignancies, CK receptor expression varies from cancer-to-cancer and its receptors influence cell trafficking into and out of the TME and aid in metastasis. The heterogeneity of cancer, in conjunction with the many gene mutations that take place during carcinogenesis and tumor growth, makes cancer treatment a formidable obstacle. Combining chemotherapeutics with RNA interference (RNAi), namely small interfering RNA (siRNA) and microRNA (miRNA), has recently emerged as an effective cancer therapy method. Targeting numerous cancer related pathways, suppression of metastasis, and overcoming drug resistance adaptations are all possible targets for combination treatments, which also have the potential for substantial benefits [62, 63]. RNAi technology has shown significant therapeutic promise for the treatment of cancer [64]. However, the administration of siRNA in vivo is restricted due to its serum instability, low cellular membrane permeability, and non-specific absorption [65]. To circumvent these constraints, delivery techniques such as liposomes, NP, chemical modification, and viral vectors have been used [66, 67]. To enhance the specificity and selectivity of tumor tissue, specialized ligands, such as monoclonal antibodies, peptides, and small molecules are often included in delivery systems [68].
CXCR4 is the CK receptor that is most often found in human cancers. CXCR4 is a G protein-coupled receptor and the biological effect of CXCR4 is caused by its binding to its specific ligand, CXCL12. For the purpose of inhibiting the CXCR4/CXCL12 axis and understanding its role in cancer, several techniques have been explored. Specific CXCR4 antagonists seemed to have the most beneficial effects. Plerixafor (AMD3100), a small molecule CXCR4 antagonist has already been given the go-ahead for clinical usage in stem cell mobilization by the Food and Drug Administration (FDA) [69]. In addition to small drugs, CXCR4 binding peptides and siRNA, which silences the expression of the CXCR4 gene, have been found to be capable of blocking CXCR4-mediated activities in treatment modalities of neoplasia [70, 71]. Besides that, suppression of CXCR4 activity by the use of CXCR4 siRNA or CXCR4 ligands is a viable option for the development of effective cancer therapeutic nanomedicines. AMD3100 is capable of functioning both as a cancer-targeting ligand for enhanced NP distribution and as a CXCR4 antagonist for the treatment of cancer [72]. In case of liver cancer and liver fibrosis, Gao et al. [73] reported the development of a series of NPs formulated sorafenib in CXCR4-targeted lipid-coated poly (lactic-co-glycolic acid) (PLGA) NPs. This was modified with a CXCR4 antagonist and sorafenib/mitogen-activated protein kinase (MEK) inhibitor-loaded CXCR4-targeted maleimide (PEG) NPs that have the potential to carry both functional siRNA and small medicines [73, 74]. Growth-regulated oncogene-α (gro-α) also known as CCL1, is released by macrophage, neutrophil, and epithelial cells, and it is involved in angiogenesis, inflammation, and wound healing [75]. In ulcerative colitis, colon adenomas, colon cancer, melanoma, breast cancer, bladder cancer, and ovarian cancer, gro-α is highly expressed [76, 77]. Overexpression of gro-α may increase the tumor cell proliferation, invasion, and metastasis [78]. Recent investigations have demonstrated that ovarian cancer tissues and sera exhibit high amounts of gro-α, while normal ovarian epithelial cells and fibroblasts express lower levels of gro-α [79]. High levels of gro-α in stromal cells induce fibroblast senescence and, subsequently, malignant transformation of ovarian epithelial cells. In addition, gro-α overexpression may accelerate the development and progression of ovarian cancer as well as the onset of endometriosis [80]. Follicle stimulating hormone 33–53 (FSH 33–53) peptide was used as an ovarian cancer-targeting agent, while siRNA targeting gro-α was utilized as a therapeutic agent. Consequently, the downregulation of gro-α inhibits the aggressive biological activity of ovarian cancer cells [81].
Absence of effective delivery mechanisms for the simultaneous administration of small-molecule medicines and siRNA/miRNA limits the performance of this combination therapy. Due to the physicochemical differences between the two kinds of agents, developing delivery methods for combinations of small molecule medicines and siRNA/miRNA is a substantial problem. Polymeric NPs have been the most effective delivery technology for drug/nucleic acid combos among the existing delivery technologies [62, 82]. A typical polymeric NP is made of a pharmacologically inert polymer that can encapsulate both classes of medicinal drugs. Examples of pharmacologically inert polymers include polyethylene, polypropylene, polyvinyl chloride, polytetrafluoroethylene, and polyvinyl acetate. List of various types of NPs loaded with different drugs for various cancer types has been included in Table 2.
List of nanomedicine for cancer therapy
NPs | Synthesis methods | Cancer type | Route of administration | Drug utilized | Important result | References |
---|---|---|---|---|---|---|
Liposomes | Liposomes can be synthesized by various methods such as film hydration method, ether injection method, and sonication method. | Breast cancer | Intravenous | Paclitaxel | Enhances the delivery of drugs to tumor sites and reduces toxicity to healthy cells. | [83, 84] |
Polymeric NPs | Polymeric NPs can be synthesized by various methods such as nanoprecipitation, solvent evaporation, and emulsion-diffusion methods. | Non-small cell lung carcinoma | Oral | Cisplatin | Enhances the delivery of drugs to the tumor sites and improves targeted therapy | [85] |
Dendrimers | Dendrimers can be synthesized by divergent and convergent methods. | Prostate cancer | Topical | Docetaxel | Enhances the delivery of drugs to cancer cells and improve targeted therapy | [86, 87] |
Gold NPs | Gold NPs can be synthesized by methods such as seed-mediated growth, reduction of HAuCl4 and citrate reduction. | Melanoma | Intravenous | Dox | Induces apoptosis in cancer cells and enhances the efficacy of chemotherapeutic drug | [88, 89] |
Silica NPs (SNPs) | SNP can be synthesized by sol-gel, Stober method, and reverse micelle method. | Pancreatic cancer | Oral | Gemcitabine | Enhances the efficacy with no evidence of local or systemic toxicity | [90, 91] |
Recently, alternate methods for delivering drug/nucleic acid combinations have concentrated on the production of pharmacologically active NPs and polymers [92, 93]. A key new target for creating combination delivery techniques for better cancer therapy is the CK receptor CXCR4 [94]. Due to its critical involvement in the progression of numerous forms of metastatic human cancer, CXCR4 is considered as an interesting target in anti-metastatic therapy [95]. To enhance cancer treatment, many CXCR4-targeted drug delivery methods, including liposomes, NPs, lipoplexes, and polyplexes, have been created [96].
Induction of immunogenicity (CK receptor/and their ligands) by myriad NM against tumor cells
Novel functionalization of potential nano-delivery systems using CKs was designed, synthesized, and characterized as silicon dioxide (SiO2) NPs. CXCL5 was covalently attached to the surface that led to potent NP internalization into CXCR2+ cells. CK-receptor binding favored the localization to specific receptor-positive cells suggesting that CKs are potential molecules for specific targeting in order to deliver cargo to immune cells [73]. Development of multifunctional NM targeting CK induction and the subsequent immune response is critically important for physiological process. Well-devised nanocarriers with optimized physicochemical properties may play a meaningful role in determining the efficiency of therapeutics. In mouse models of melanoma, a combination of PLGA NPs embedded in CXCL1 hydrogels showed superior tumor inhibition capability via dual advantage of neutrophils phagocytosis in addition to targeted delivery of CKs [97]. An injectable hydrogel system stimulates tumoricidal immunity following surgical resection of glioblastoma multiforme (GBM) which mitigates its relapse. The hydrogel consists of tumor-homing nanoregulator, which induces ICD, suppressing IDO1, and CXCL10, for persistent T cell infiltration. Following delivery in the resected tumor milieu, the hydrogel provided a “hot” tumor-immunity niche for eliminating tumor cells and suppressing postoperative GBM recurrence significantly, and augmented the survival of mice with postoperative intracranial tumors [98]. CCL2-coated liposomes with an encapsulated oncolytic adenovirus (immunodominant/presentation peptide trimer-early region 1A protein) [Ad(I/PPT-E1A)] helps in recruiting preferentially, CCR2 expressing circulating monocytes in the vicinity of prostate tumor. This method of delivery does not induce complex-related toxicities following incubation with cancer cells and did not affect the oncolytic activity of virus in vitro. Intravenous administration of this nanoformulation results in significant reduction in tumor volume and pulmonary metastasis in tumor-bearing mice compared with empty virus vector Ad(I/PPT-E1A) [99]. The development of cationic NPs (CNPs) promotes the cytotoxic potential of primary NK and human NK-92MI cells against triple-negative breast tumor cells compared with the control NK cells. CNP modulated the expression of CK receptors CCR4 and CXCR4 in NK cells. CNP-treated NK cells significantly inhibited tumor formation in the animal model of triple-negative breast cancer. Additionally, CNP-treated NK cells were tracked efficiently by magnetic resonance imaging in vivo, indicating successful CK-mediated NK cell-based tumor immunotherapy in breast cancer [100].
The CCL2 and its cognate receptor CCR2 (CCL2/CCR2) axis are critical in fostering and maintaining TME by recruiting immunosuppressive myeloid cells including the TAMs, and thus it presents an opportunity to exploit diagnostic and therapeutic purposes. Pancreatic ductal adeno-carcinoma (PDAC) is currently considered as a deadly malignancy with poor prognosis due to aggressive biology, lack of effective tools for diagnosis at an early stage, and limited treatment options. CCR2 targeting novel ultrasmall copper NPs (Cu@CuOx) as nano vehicles was engineered for positron emission tomography imaging by intrinsic radiolabeling with 64Cu and also for the delivery of gemcitabine in the mouse model of PDAC. Systemic administration of Cu@CuOx, loaded with gemcitabine and targeting CCR2, suppressed growth and the progression of PDAC tumor in a syngeneic xenograft, established in mouse. The therapeutic strategy prolonged the survival and served as an image-guided therapeutic agent with great potential for translational benefits [101]. In a mouse (C57BL/6) tumor model of MCA-203 fibrosarcoma, co-encapsulated CKs (CCL2) and polynucleotides (miR-142-3p) polyarginine nanocapsules (NCs) induced a potent monocyte-macrophage chemoattraction that could be used to direct the therapy to these cell subsets. Encapsulated CCL2 in the micellar aqueous domain of the lipid core of the NC attracts MDSC. This multilayer polymer-based NC can serve as a vehicle for the co-delivery of both RNA and proteins and are promising candidates as nanomedicines for the modulation of MDSC [102]. A simple thiol disulfide exchange conjugation strategy was utilized to prepare reducible polymeric AMD3100 (rPAMD)-single-stranded (SS) siRNA polyplex conjugates. These conjugate polyplexes were prepared using thiol-terminated siRNA and a bio-reducible branched polycationic inhibitor of the CXCR4 CK receptor (rPAMD). The polyplex conjugates were tested in B16F10-luciferase (luc) mouse melanoma cells. Using polycations which inhibit CXCR4 these polycations retain their inhibitory activity as suggested by the strong ability to prevent invasion of cancer cells. With systemic anticancer and anti-metastatic therapies [103]. In a study with human macrophages, arginine-rich polypeptide complexed polyinosinic:polycytidylic acid [poly(I:C)], enveloped with anionic polymeric layer nanocomplexes, significantly increased the cytotoxic ability of the treated macrophages against the cancer cells. Macrophages treated with the nanocomplexes have no detrimental effect on its viability and efficiently stimulate the secretion of the T cell recruiter CKs CXCL10 and CCL5, for an effective anti-tumor immune response [104]. In a solid tumor model of lung cancer, it is demonstrated that a protocol for the pulmonary delivery system, based on perfluoro carbon nano emulsions for dual delivery of a partially fluorinated polymeric CXCR4 antagonist and anti-signal transducer and activator of transcription 3 (STAT3) siRNA [105]. CXCR4 and STAT3 are critically important for the proliferation, angiogenesis, and metastasis of tumor cells. The decorated nanoemulsion inhibited CXCR4 and STAT3, documented by effective anti-invasive ability in vitro and related anti-metastatic activity in vivo. The combined nanoemulsions showed a comprehensive tumoricidal effect in lung metastasis of 4T1 breast carcinoma model involving apoptosis, anti-invasive activity, anti-angiogenic effect, and overcoming of the immunosuppressive TME [82]. A multifunctional polycation was synthesized to demonstrate their ability to inhibit CXCR4 and systemically deliver siRNA to treat different stages of metastatic breast cancer. Dual inhibition of STAT3 and CXCR4 prevents primary tumor growth and metastasis via multifaceted mechanisms of the polyplexes depending both on local and systemic effects. Besides breast cancer, other types of cancer also respond to this therapy. Pharmacokinetic analysis of the construct demonstrated reduction in the rate of renal clearance of the polyplexes due to fluorination and allows greater accumulation in the tumor [83]. CXCR4-targeted delivery system employing doxorubicin-loaded mesoporous SNPs, capped with an azide-containing modified T22 peptide by click chemistry. Tyrosine 5 (Tyr5), Lys7, and Tyr12 residues in T22 peptide significantly increased the affinity for the CXCR4 receptor, overexpressed in non-Hodgkin’s B-cell lymphoma. The pores in SNPs were capped by the peptide to prevent the release of the drug and facilitated the uptake via the CXCR4 receptor. T22 peptides were degraded by lysosomal proteolytic enzymes and allowed intracellular doxorubicin release [106]. Several others have reported that LFC131 deoxy-tyrosine-arginine-arginine-2-naphthylalanine-glycine (d-Tyr-Arg-Arg-2-Nal-Gly), a low molecular weight CXCR4 peptide antagonist, conjugated to Ocarboxymethyl chitosan or PLGA NPs enhanced the targeted delivery of doxorubicin and docetaxel in CXCR4 overexpressing lung cancer cells [107, 108]. CXCR4 binding peptides have been used to improve nucleic acid delivery with cationic peptides and cationic polymers. CK-derived peptides have been used as carriers for gene delivery [109]. Acyclam derivative FDA-approved CXCR4 antagonist AMD3100 (plerixafor) has been reported to bind and inhibit CXCR4 signaling in several animal models and also in clinical trials [110]. AMD3100 was also used in association with the multi-compartment PLGA NPs in order to target CXCR4 overexpressing breast tumor cells [111]. The targeted NPs were specifically picked up by CXCR4 overexpressing neoplastic breast tumor cells, and thus effectively blocked CXCR4 signaling. The PAMD polymers demonstrated dual functionality for delivering nucleic acid (gene and siRNA) delivery vectors plus CXCR4 antagonists and inhibiting metastasis of tumor cells in several experimental models in vivo [112, 113]. The hydrogels were loaded with the CCR7 ligand CCL21 and were injected subcutaneously in a murine model of cancer. CCL21-loaded hydrogels attract DC preferentially at the treatment sites compared with non CCL21-loaded hydrogels, enabling immunomodulation in cancer [114]. Robert Langer group has designed shear-thinning and self-healing polymer NP hydrogels, working as a tunable and injectable biomaterial platform for the recruitment of DCs [114]. In a preliminary study, CCL21 packaged vault NPs were designed as a feasible preclinical treatment option against GL261 cell-induced glioblastoma, developed subcutaneously in the left flank of C57BL/6 mice. CCL21 packaged vault NPs demonstrated reduction in tumor volume change (−21.12%) including downsizing in average tumor mass to 9.91 mm3 compared to 93.37 mm3 in untreated control group [115]. In an orthotopic genital tumor model of mice, self-assembled nanofiber elicits a robust antitumor immune response mediated by local mucosal immunization. Self-assembling nanofibers are a powerful antigen delivery technology for human papillomavirus (HPV)-related tumour vaccines and have the potential to induce strong anti-tumor cellular immunity that is specific to the HPV antigen [116]. Immunization with these nanofibers in a genital TC-1 tumor in mice via intravaginal, intranasal, or subcutaneous route elicits systemic immune response with the generation of antigen specific CD8+ cytotoxic T cells, producing significantly higher IFN-γ or granzyme B, besides reducing and restricting tumor infiltrated immunosuppressive Treg cells and MDSCs. This treatment also induces the production of CXCL9 and CXCL10, and expressing CXCR3+ CD8+ T cells in tumor tissues suggesting improved effector T cell functions in the TME [117]. Conjugation of CK CXCL13 to nanoencapsulated rituximab as a targeting ligand in lymphomas further enhanced the tumoricidal potential in xenografted humanized non-obese diabetic (NOD)-SCID-gamma-hemolytic complement (NSG-Hc1) mice by augmenting anti-lymphoma cellular responses. Conjugation of CXCL13 over the surface of nanoencapsulated rituximab results in efficient targeting of lymphoma in the brain [118]. Zhao et al. [119] devised a new method delivering an immune active NP called erythrocyte-anchored systemic immunotherapy (EASI), capable to colocalize with the metastatic lung cancer cells following dislodging from the erythrocyte surface. This method controls the progression of metastasis and develops an adaptive response for systemic tumor suppression. This NP called ImmunoBait, encapsulating the CK CXCL1 is anchored on the surface of erythrocytes and is capable to attract effector immune cells [119]. Targeting tumor associated adipocytes in the TME of breast cancer involving CCL2 may provide an opportunity to engineer new particles. Liu et al. [120] showed, plasmid DNA encoding the CCL2 trap and delivered by lipid-protamine-lipid NP induce enhanced therapeutic efficacy in triple-negative breast cancer by significant inhibition of tumor growth in the TME. This method could prevent the CCL2-mediated recruitment of monocytes/macrophages which transformed into myeloid suppressor cells in the TME. The CCL2 trap also suppresses adipocytes, recruits T cells and downregulates immunosuppressive and disease-promoting M2 macrophages [120]. Many facets of the cancer therapeutics involving duets of different NM and CKs has been summarized in Figure 5.
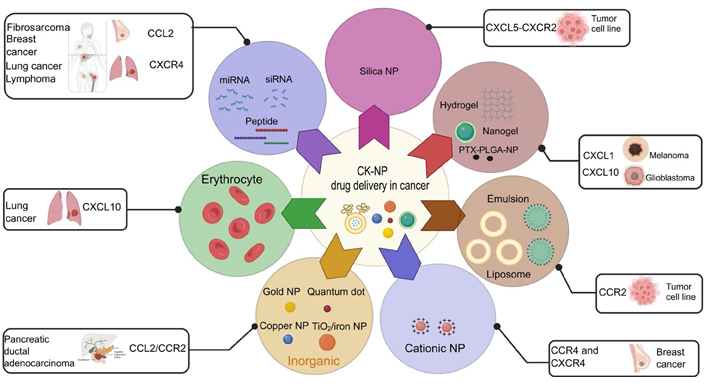
Various NM and CK-aided cancer immunotherapy. Immunogenicity by CK receptors and their ligands against tumor cells was studied using different kinds of nanodelivery procedures in experimental animal models of cancer
CK secretion by DC/monocytes following exposure to drug-loaded NP
DCs are antigen-presenting cells that connect innate and adaptive immunity. In nonlymphoid tissues, immature DCs capture antigens. DCs mature in response to allergens, inflammation, pro-inflammatory cytokines, and bacteria. This process leads to antigen processing, increased expression of major histocompatibility complex (MHC), co-stimulatory molecules, upregulation of CK and cytokine receptors, and cytokine and CK production. Mature DCs move to lymph nodes to activate T lymphocytes. Modulation of DC functions may be determined by the size of the NP. In vivo, for instance, lung DCs catch 20 nm polystyrene (PS) particles more often than 1,000 nm PS particles [121]. In vitro, 20 nm PS particle doesn’t affect viability, maturation markers, or antigen uptake in murine bone marrow-derived dendritic cells (BMDCs), however, it significantly downregulates antigen degradation in a size-dependent manner, accumulates in lysosomes, but does not affect T-cell proliferation [121]. It seems that the trafficking of NPs and materials to the draining lymph nodes is size-dependent. Indeed, only tiny particles (20–200 nm) may readily drain to the lymph nodes [122]. Carbon black NPs upregulate CD86, CD80, and MHC-II expression on murine BMDCs, which is responsible for increased allogenic mixed lymphocyte response [123]. Titanium dioxide (TiO2) NPs have also been shown to enhance the expression of CD80, CD86, MHC-II, and TNF-α in murine BMDCs [124]. Ultrafine SNPs lowered cell survival, induce minor phenotypic alterations, and dramatically increased TNF-α generation in murine BMDCs and DC line DC 2.4 in a size-dependent manner [125]. Notably, these effects were associated with in vivo inflammatory response in C57BL/6 mice, injected with liquid matrigel containing SNPs when given subcutaneously [125]. Amorphous SNPs were able to alter cell survival through apoptosis and stimulate partial maturation of BMDCs, demonstrated by the increased expression of co-stimulatory molecules and MHC-II and also activate NOD-, LRR- and pyrin domain-containing protein 3 (NLRP3) inflammasome [126]. These findings demonstrated that some NPs may increase DC maturation and activation, resulting in T lymphocyte activation (Figure 6). M1 macrophages induce unfavourable impact on inflammation caused by large macrophage populations at the lesion site. In addition, macrophages can swiftly migrate to tumor sites, release CKs, and enable them to deliver drugs precisely. The immune system’s capacity to target and eliminate tumors marked a paradigm change in cancer therapy which was previously treated with cytotoxic drugs [127]. Despite immunocompetent cells with intrinsic anti-tumoral capacity, tumor cells produced immunosuppressive signals to generate immune tolerance and facilitate tumor development [128]. Among the several cells engaged in this event, TAMs are crucial actors with the ability to enhance cancer cell proliferation, angiogenesis, and metastasis [129]. TAMs have anti-inflammatory and tolerogenic properties comparable to M2-like macrophages [130]. Importantly, recent research has suggested that reprogramming TAMs into pro-inflammatory and tumoricidal M1 states might be a potential method for reactivating immunity against the tumors [131, 132]. The use of drugs that activate TLRs has been a key development for skewing TAMs into M1 phenotypes [133]. TLRs activate myeloid differentiation primary response 88 (MyD88) and TIR-domain-containing adapter-inducing IFN-β (TRIF) pathways when they engage with their respective agonists, resulting in enhanced immune responses [134].
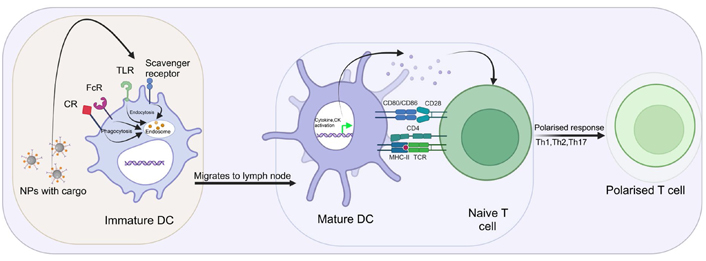
Interaction of NMs with DC. Immature DC receptor helps in aggregation, endocytosis, and phagocytosis of NPs. Protein aggregates are processed by DC to deliver MHC-II-linked peptides to naive T lymphocytes. This contact may be a signal of danger, leading to maturation and gene transcription. After maturation, DC may produce cytokines and CKs which activate and polarise naive T-cells. CR: complement receptor; FcR: Fc receptor; →: upregulation; : upregulation
Emerging danger signals created by compounds, including NMs, can either activate DCs directly or indirectly causing tissue harm. Thus, it is hypothesised that immune cells might detect NMs, labelled as NP-associated molecular patterns, similar to infections (Figure 6) [135]. Nano TiO2 and nano SiO2 particles activate the formation of NLRP3 inflammasome in THP-1 cells, which correlates with in vivo lung inflammation necessitating IL-1 receptor expression [136]. Activation of inflammasome by nano TiO2 and nano SiO2 particles would occur via release of ATP and adenosine receptor signaling [136, 137]. Furthermore, 30 nm SNPs trigger intracellular ATP release, and P2RX7 signaling resulting in ROS formation, inflammasome activation, and stimulation of IL-1 and IL-18 secretion in lipopolysaccharides (LPS) matured murine BMDC [138].
Role of different groups of CK-loaded NPs in tumor therapy: implication of lymph node for immune response
The host’s lymphoid organs and tissues serve critical functions in protecting the host against infections and tumor spread. A range of host cell types participate in the immune response, generated by microbial or tumor antigens. One of the most essential aspects of this response is a complex process of direct leukocyte trafficking that is controlled in part by chemotactic communication system based on CK interactions with cognate cellular receptors [139]. Lymphatic veins are present throughout the body and carry extracellular fluid and solutes to draining lymph nodes and the systemic circulation. New study shows that lymphatic tubes actively transfer molecules and modulate the immune system. Immunomodulatory drugs are delivered to lymph nodes through lymphatic channels to affect immune responses. The size of NPs is one of the first characteristics studied to optimise lymphatic medication delivery. After intradermal tail injection, 20–100 nm fluorescent PEG-stabilized poly (propylene sulphide) NPs were accumulated in the lymph nodes. Importantly, using fluorescent NPs, scientists were able to observe the lymphatic network of the mice [140]. Following injection of pH-sensitive lipid NPs subcutaneously into the inguinal areas of mice, Nykänen et al. [141] noticed a similar occurrence. It was observed that 30 nm formulation of solid lipid NPs were more efficiently delivered and distributed in lymph nodes and are grabbed by CD8+ DCs compared to bigger size (100 nm and 200 nm) formulations. Immune cells in peripheral lymphatic channels travel to local lymph nodes and use the lymphatic system as a highway. DC, monocytes, neutrophils, and lymphocytes (B and T cells), move along the CCL21-CCR7 axis, which is facilitated by lymphatic channels [142]. CCR7 helps immune cells migration to lymph nodes and directs DC to lamina propria and also to lungs and skin. CCR7 regulates DC-lymphatic vessel connection. DCs also enhance the lymphatic channel permeability [142, 143].
Chemotaxis is used by macrophages, DC, and neutrophils to transport and present antigens to secondary lymphoid organs. Numerous pathogens have developed complex strategies to evade the host’s immune response, including the inhibition of leukocyte chemotaxis towards the infection areas [144]. Creation of novel vaccination adjuvants, anti-tumor reagents, anti-inflammatory and anti-microbial therapies may be influenced by the development of therapeutic techniques to restore and reliably control the chemotaxis of the immune cells. Locally engineering CK gradients to promote the accumulation of critical immunological players, such as DC and neutrophils, or to induce cascades of immune responses to eradicate infections or malignancies is a potential method [145, 146]. Recent developments in nanotechnology have opened the door for novel approaches to achieve this objective by using controlled release of materials to construct CK gradients for use in both fundamental research and therapeutic applications [147, 148]. In vitro migration of DC, monocytes (DC progenitors), and T cells has been shown to be directed by biodegradable NPs that provide a prolonged release of different CKs [148, 149].
Future perspectives
Emerging realms in the areas of nanotechnology, tailoring different NMs have revolutionized understanding in health and disease. Although still in its infancy, it is considered that combining current trends in biological engineering, cell therapy, nanotechnology, and material chemistry can result in novel therapeutics based on the immune cells. This endorses extremely diverse platforms with great therapeutic potential for superior personalized diagnostics and abatement of the disease. In this review, we have tried to focus on many aspects of NMs and CK and their role in developing novel therapeutic platform for benefits in fighting against cancer. NP encapsulating drugs should be carefully evaluated as both drugs and the NP could be toxic to patients. Such toxicities could result from a long-term accumulation of NPs inside the body, which significantly complicates clinical applications of NPs compared to free drug treatment. Therefore, the use of bio-degradable materials for the fabrication of NP is quite appealing. With reference to vaccine development, induction of long-lasting immunological memory by NPs should be emphasized, as it is the key to achieving sustained immune rejections against both pathogens and cancer. Future NP engineering should address the above issues to facilitate clinical and translation benefits of NPs. The NP approach, featuring safe and effective immune inductions will continue to retain a crucial position in the development of next-generation vaccines. Engineering novel NP having desired impact on adaptive and innate immune system for the efficacy of the therapy is the prime objective of its application. The pivotal role of immune response, in particular, the adaptive immune response governs and meticulously provides an efficient and effective response to foreign, nonself object like NM inside the human body. Despite clear growth in the nanosafety field, understanding the impact of NMs upon the innate and adaptive immune system needs in-depth study.
Specific examples of these knowledge gaps relate to the following issues:
Precise manner in which NMs interact/bind with key innate immune cells like NK cells and DC. Receptor-mediated interaction, NMs interaction with specific PRRs on specific innate immune cells, governing the entry mechanism and signaling cascades that might be relevant to processing of NM by the cells are important.
Impact of NM on different physicochemical characteristics of cells with phagocytic potentials including macrophages, neutrophils, and DCs.
Physiological response with reference to structure and functions of adaptive immune cells like T and B lymphocytes and granulocytes including eosinophils, basophils, and mast cells.
Understanding of tripartite or multipartite interaction of more than one immune cell and NP in different organs. Effect of NP exposure and consequent understanding of dynamic, fluid-flow systems for gathering information on in vivo scenarios is required, ostensibly mediated via multicellular participation.
Comprehensive understanding of the complete signaling cascade under NP exposure involving cytokine and CK-mediated events based on concentration and duration of the NP exposure. Also, it is urgent to assess the effects of NP exposure involving cytokine and CK on cell/tissue/organ structure and functions for quantitative and qualitative evaluation.
Further research is needed to develop novel NMs with catalytic properties responsible for the induction of ICD, endoplasmic reticulum (ER) stress, and ROS generation. Understanding of the DAMPs and cytokines/CKs is critically important for immunogenicity in dying cancer cells.
For many nanoformulations, coating the surface of NPs with PEG (PEGylation) is required to obtain increased circulation half-life and accumulation in the tumor bed. However, PEGylation caused chronic-foot syndrome, generating anti-PEG antibodies which could delay clinical application. Consideration of the alternative or refining of the strategy is necessary.
Research is needed to develop strategic NM which could induce apoptosis, necroptosis, or ferroptosis type of cell death. The methodology needs to be synchronous and selective for killing of the cancer cells. Future studies may provide necessary insights for the development of novel treatment strategies to increase the ICD by using NMs and validate these observations in clinical settings. This could be an exciting area for upcoming research.
Requirement of effective and stringent regulations in NM safety and regulatory measures for the newly introduced materials is a critical issue in pre-clinical and clinical trials with reference to the modalities of ICD. Challenges remain in many areas including the induction of increased immunogenicity of dying (dead) tumor cells in anti-cancer therapy by nanoformulations.
Concern on natural and synthetic molecules used for fabricating NPs with reference to properties like biocompatibility and biodegradability which also modulate ICD. Attention needs to be given to the various organic and inorganic constituents of the nanocarriers.
Requirement of greater insight in physical properties including size, charge, functionality and surface properties, etc. of NPs and their interaction with the dying malignant cancer cells with reference to phagocytosis, antigen presentation.
It is imperative that more studies should be focused on connectivity and interdisciplinary aspects of immunooncology, NMs and nanotechnology in order to drive further progress in the areas of cancer therapeutics. It will allow elucidation of vital information on detrimental and beneficial effects of NPs exposure on host defence mechanisms and inform realistic low-dose, long-term, repeated exposure scenarios. NPs are promising however, compatibility issues, especially long-term toxicity, are the drawbacks for certain systems, especially nonbiodegradable ones.
Conclusions
CKs and their receptors are important for immune system modulation and cancer immunotherapy. NP surface-mediated sequestration reduces inflammatory CK upregulation and drives selective cell targeting for imaging and delivery. Interdisciplinary cooperation will uncover fresh concepts for future therapeutic options, based on CK-NP interactions. Treg cells, TAMs, and TAA expression may be important for immunotherapy and immunosurveillance. Unique properties and activities of biological NPs increase the effectiveness of immunotherapy and may act as an ICD inducer. NPs are immunostimulants and work as an adjuvant, capable to deliver peptides, specific antigens, or whole cell membranes. NP-targeted checkpoint inhibitors can also reverse TME immunosuppression. To pick the appropriate combinational strategy, there should be distinct nanoplatforms for different tumors, depending on the limitations of diverse treatment modalities and TME complexities. To improve tissue localisation, cell permeability, and anti-cancer immune response following systemic delivery, NP systems with high tumor accumulation and scalability must be completely exploited. Since cancer is a chronic inflammatory disease, making highly effective targeted nanoplatforms for ICD will reduce drug resistance and side effects. It will also activate immune cells to kill more cancer cells and make more proinflammatory cytokines/CKs in the TME. Cancer immunotherapy faces several challenges which include inadequate understanding of the disease state, TME, and the absence of truly mimicking animal models. Regulating the CK network in TME via NP could fine-tune potential pharmacological targets, migration of leukocytes, and synergistic interactions for major breakthroughs in therapy. Nanomedicine in health care needs better knowledge of NP’s behaviour in biological environments, precise characterization to improve repeatability, and greater clinical use which may advance immuno-oncology and cancer immunotherapy.
Abbreviations
anti-CD47: | anti-cluster of differentiation 47 |
ATP: | adenosine triphosphate |
BMDCs: | bone marrow-derived dendritic cells |
CCL14: | C-C motif chemokine ligand 14 |
CCR1: | C-C motif chemokine receptor 1 |
CKs: | chemokines |
CNPs: | cationic nanoparticles |
CRT: | calreticulin |
CXCL1: | C-X-C motif chemokine ligand 1 |
CXCR3: | C-X-C motif chemokine receptor 3 |
DAMPs: | damage-associated molecular patterns |
DCs: | dendritic cells |
gro-α: | growth-regulated oncogene-α |
HMGB-1: | high mobility group box 1 |
ICD: | immunogenic cell death |
IFN-γ: | interferon-gamma |
IL-1: | interleukin-1 |
MDSCs: | myeloid-derived suppressor cells |
MHC: | major histocompatibility complex |
miRNA: | microRNA |
NCs: | nanocapsules |
NK: | natural killer |
NMs: | nanomaterials |
NPs: | nanoparticles |
PDAC: | pancreatic ductal adeno-carcinoma |
PEG: | poly(ethylene glycol) |
PLGA: | poly (lactic-co-glycolic acid) |
PS: | polystyrene |
ROS: | reactive oxygen species |
SiO2: | silicon dioxide |
siRNA: | small interfering RNA |
SNPs: | silica nanoparticles |
STAT3: | signal transducer and activator of transcription 3 |
TAAs: | tumor-associated antigens |
TAM: | tumor-associated macrophage |
TiO2: | titanium dioxide |
TLR4: | Toll-like receptor 4 |
TME: | tumor microenvironment |
TNF-α: | tumor necrosis factor-alpha |
Treg: | regulatory T |
Tyr5: | tyrosine 5 |
Declarations
Acknowledgments
RS thanks Indian council of medical council research (ICMR), India; No. 2021-12946/CMB-BMS for senior research fellowship. The Figures were created with BioRender software (https://biorender.com/).
Author contributions
RS, PS: Writing—original draft, Writing—review & editing. PPM: Conceptualization, Supervision.
Conflicts of interest
The authors declare that they have no conflicts of interest.
Ethical approval
Not applicable.
Consent to participate
Not applicable.
Consent to publication
Not applicable.
Availability of data and materials
Not applicable.
Funding
This work was supported by IOE grant, No. R/Dev/D/IOE/Incentive/2021-22/32275 to Partha Pratim Manna by Banaras Hindu University (BHU). The funders had no role in study design, data collection, and analysis, decision to publish, or preparation of the manuscript.
Copyright
© The Author(s) 2023.