Abstract
Vagus nerve stimulation (VNS) has gained prominence in the treatment of various clinical disorders such as migraine, depression, and tinnitus. Based on increased scientific knowledge of the VNS and insights into the vagus nerve (VN) function and anatomy/conduction, robust treatment approaches have been developed. There are both noninvasive and invasive VNS (iVNS) techniques. Currently, only iVNS techniques are approved by the US Food and Drug Administration (FDA). In contrast, transcutaneous VNS (tVNS) is a new treatment option that is receiving increasing attention. The tVNS application uses the cutaneous distribution of afferent VN fibers in the auricle, the auricular branch of the VN (ABVN), or in the neck, the cervical branch of the VN (CBVN). However, the tVNS technique has not yet been sufficiently researched in its application and mode of action to be used clinically on a large scale. Moreover, the stimulation parameters of the VN vary widely in different studies. Despite the growing number of research papers on this topic, more coherence in neurostimulation research and neuroanatomical basis is needed. The aim of this review is to highlight new clinical treatment options based on existing clinically applied treatment options. In this article, current clinical applications of tVNS are analyzed and important stimulation parameters are highlighted. Based on this data, useful new tVNS therapies are recommended. The focus will be placed on the study of inflammatory processes associated with cancer and on applications to cardiovascular events such as heart failure.
Keywords
Vagus nerve, transcutaneous vagus nerve stimulation, inflammation, cancer, heart failure with preserved ejection fractionIntroduction
Vagus nerve stimulation
In conjunction with basic research, vagus nerve stimulation (VNS) has been investigated as a second or third-line therapy for a number of clinical conditions, including migraine and tinnitus [1, 2]. Several VNS treatment strategies have demonstrated significant antidepressant and seizure-inhibitory effects in clinical trials [3, 4]. Today, there are Food and Drug Administration (FDA) approvals in the United States for invasive VNS (iVNS) in chronic epilepsy and pharmacoresistant depression.
To truly understand the principle of transcutaneous VNS (tVNS), it is important to understand the function of the autonomic nervous system (ANS), particularly the vagus nerve (VN). The ANS consists of sympathetic and parasympathetic divisions, with the VN being the main component of the parasympathetic nervous system.
Through this, the ANS influences the function of a number of end organs, glands, and involuntary muscles throughout the body.
Nowadays, there are invasive (surgically implanted) and non-invasive (transcutaneous) techniques of VNS.
Given dedicated clinical trials, only iVNS has been approved by the FDA. In 1997, iVNS was approved as a treatment option for refractory epilepsy. In 2005, the same device was approved by the FDA for chronic treatment of resistant depression [5, 6].
The perioperative risks and postoperative consequences argue against treatments with iVNS devices. Therefore, they have been limited to the treatment of severe and intervention-resistant cases only. Potential side effects of surgery include peritracheal hematoma due to surgical trauma and other respiratory complications such as dyspnea and vocal cord dysfunction. Another complication may be bradyarrhythmia during device placement. In addition, iVNS may exacerbate obstructive apneas and hypopneas or cause changes in breathing patterns during sleep [7, 8]. These potential side effects limit its applicability to patients who do not respond to conventional therapeutic strategies. For these reasons, iVNS is only suitable as an additional, second, or third treatment option.
Therefore, tVNS as an alternative method to iVNS seems to be a new treatment option that can overcome these limitations and even be considered as a possible first-line therapy. This is shown by the first recent studies, which achieve equivalent results to iVNS but are associated with fewer risks [9, 10].
A major difference between tVNS and iVNS is that signals travel across the nucleus tractus solitarii (NTS) and bilateral efferent impulses are delivered to the cardiac surface.
Adverse cardiac events therefore seem very unlikely because asymmetrical stimulation of the efferent motor fibers of the heart is not expected [11].
In contrast to the previous surgical application, this new technique is based on the application of electrical currents via surface electrodes. These usually target the auricular branch of the VN (ABVN) and the cervical branch of the VN (CBVN), which are both lateral branches of the VN [12].
The current review, elucidates the pathophysiology of the brain-heart axis that underlies the relationship between hypertension and “heart failure (HF) with preserved ejection fraction” (HFpEF) and the rationale and evidence for therapeutic neuromodulation in HFpEF by tVNS in addition to pharmacologic treatments.
Transcutaneous forms of VNS
Transcutaneous cervical VNS
One option for transcutaneous stimulation is CBVN. The CBVN that sensitively innervates the skin of the neck is used as a target for some tVNS devices (e.g., gammaCore®, electroCore LLC). The skin surface electrodes of these wearable devices are placed on the neck to stimulate the afferent fibers of the CBVN noninvasively [13–15].
This form of tVNS treatment is now FDA-approved for acute treatment and prevention of episodic cluster headache and for acute treatment of migraine [16]. One drawback is the thick skin barrier in the neck, which requires relatively strong currents to overcome the natural resistance of the skin and achieve optimal results.
Although transcutaneous cervical VNS (tcVNS) appears to be beneficial at first glance, it is not free of undesirable effects. In particular, the stimulation fields in the neck are diffuse, so that non-vagal nerves or efferent cervical fibers may be co-stimulated. In addition, everyone’s skin barrier is hugely different depending on their constitution. This makes accurate stimulation difficult. Commonly observed side effects include neck pain, nasopharyngitis, oropharyngeal pain, vomiting, dizziness, skin irritation on the stimulation site, or sensitivity to the conducting gel [13, 17].
To achieve the greatest results in threshold activity and an adequate stimulation, the effect of tissue types on stimulation was analyzed. Finite Element Method, a magnetic resonance imaging (MRI)-derived method was used to analyze cellular components activated with tcVNS. To obtain more scientific information on activation thresholds and electric field changes, different tissue types, both macroscopic (skin, muscle, remote) and mesoscopic (nerve sheath, cerebrospinal fluid), were an essential part of the study of Mourdoukoutas et al. [18].
Clinical tests demonstrated that activation stimulation is directly influenced by deeper tissues and that tissue conductivity has effects on polarization of axon membranes. According to this model, it can be assumed that tcVNS recruits A and B axon fibers but not C fibers [18].
Transcutaneous auricular VNS
The VN has a cutaneous representation in the “Ramsay-Hunt zone” located in the auditory canal. Via the Wrisberg intermediate nerve, cutaneous stimuli reach the NTS nucleus, which is the main brain area for integration of vagal afferents in the brainstem [2, 19]. Stimulation of the “Ramsay-Hunt zone” has shown positive effects on seizure control [20]. Marked vagus-evoked potentials have even been observed after stimulation in the tragus [21]. The tVNS is a non-invasive, simple emergency treatment with few side effects that has spread worldwide by stimulating the ABVN in the tragus [22].
ABVN is a receptive field of afferent VN fiber endings, which can be non-invasively stimulated via surface skin electrodes on the outer ear [23]. These primarily afferent fibers flow to the NTS. Stimulation of the afferent fibers is achieved by applying two surface electrodes (e.g., NEMOS® Cerbomed GmbH, tVNS® tVNS Health GmbH) on the outer ear. The most often seen target is the cymba concha (Figure 1) [23–25].
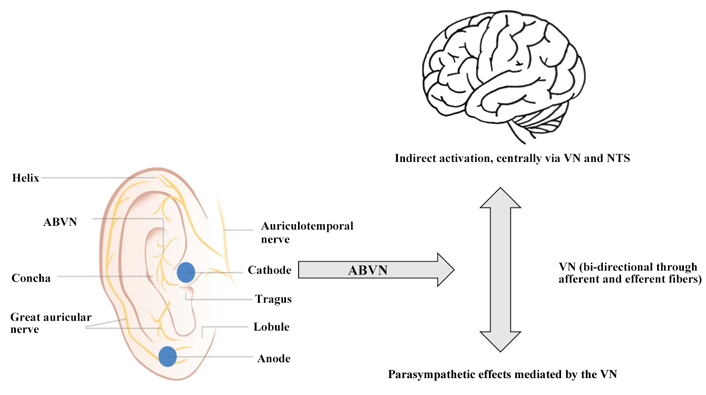
Possible mechanism for parasympathetic activation via ABVN. Activation of the vagal afferents of the ABVN leads to the stimulation of the central nervous system via the NTS and indirectly to the main bundle of the VN, which includes both afferent and efferent fibers. This process is represented by the grey arrows
The large surface of the electrodes yields makes it difficult to recruit only vagal afferents of the VN, and not non-vagal auricular nerves. The implications still remain controversial [26]. Nevertheless, ABVN stimulation is considered as safe [27]. Only minor side effects, like headache, skin irritation at stimulation sites, and dizziness are expected [17, 28].
The conduction of the ABVN is described in an article by Peuker and Filler in 2002 [29]. An anatomical dissection of the human ear presents the diffuse innervation of the ear. Currently, most researchers refer to this article because there is still a lack of comprehensive data about the innervation of the ear.
Nonetheless, the cymba concha is thought to be innervated exclusively by the ABVN. Other regions, such as the tragus and the posterior and inferior walls of the auditory canal, are also thought to be regions for the activation of vagal afferents [24].
Based on this assumption, different ear targets and electrode placement techniques for transcutaneous auricular VNS (taVNS) have been outlined in laboratories [24, 30].
Targeted fiber types
In order to better understand the principle of iVNS and tVNS, it is necessary to analyze the structure of the VN. Only with better understanding can maximum effectiveness and reliability in therapy be achieved. The VN fibers can be classified into three groups based on their diameter: group A (Aα, Aβ, Aγ, and Aδ), group B, and group C. The difference among these groups is determined by myelination thickness and different diameters. This leads to different conduction velocities. Accordingly, thicker myelination has a positive effect on conduction velocity and signal propagation.
A-group fibers are thick and myelinated. They possess afferent and efferent qualities. They can be found in both motor and sensory pathways. Typically, these fibers have a diameter of around 1–22 µm and a conduction velocity of 3 m/s to 120 m/s.
B-group fibers are moderately myelinated and less thick, with diameters of about 1–5 µm and conduction velocities ranging from 3 m/s to 15 m/s.
Lastly, C-group fibers are not myelinated and have the thinnest diameters 0.4–2 µm and a conduction speed of 0.5 m/s to 2 m/s [31].
CBVN is made up of 80% unmyelinated C-fibers and about 20% myelinated A- and B-fibers [32]. Today’s hypothesis is that the therapeutic effects of CBVN are attributed to the maximal recruitment of A- and B-fibers, even with full destruction of C-fibers [33].
Results of several trials showed low side effects during stimulation, which leads to the assumption that the therapy is well-tolerated [34]. Additionally, a study by Stefan et al. [1] showed that patients showed no signs of pain while being treated with tVNS. This suggests that there is no activation of afferent C-fibers or thin Aδ axons [1].
Tests of tVNS devices with a current of 10 mA support these conclusions. The activation of A- and B-fibers was observed, but that of the thin, unmyelinated C-fibers was not. This is likely a result of the diameter of the fibers [18].
In contrast, the distribution of nerve fiber types in ABVN has not been as thoroughly studied as in CBVN. For this reason, the presence of nerve fiber types remains speculative, and effective results must be evaluated based on subjectively perceived therapeutic benefit.
Despite positive effects of different parameters, such as frequency or impulse on various structures of the brain, having been discerned, it is difficult to declare generally valid stimulation parameters. Due to individual benefits in VNS and differences in adapting to VNS, stimulation parameters must still be selected individually for each patient and stimulation parameters.
Stimulation parameters
Recently, some investigators have produced critical reviews of technical information about tVNS treatment (e.g., stimulation site, tVNS device, electrode design, intensity, frequency, waveform parameters). The collection of this information is intended to help achieve better outcomes in the use of tVNS and to develop a checklist for reporting studies so that further studies can be guided, and results can be better understood.
The evaluation of stimulation parameters, based on the research work of Yap et al. [31] and Verma et al. [35], is presented in Table 1. For clarity and because of the review’s focus on cardiovascular disease, only studies associated with this topic were selected. The listing of all relevant studies was evaluated from a technical point of view and presented in Table 2. The initial review showed that the performance of both the active and sham groups was quite different in the studies. In the active arm, the interquartile range (IQR) of stimulation intensity was 0.85–10.0 mA, stimulation frequency was 20.0–25.0 Hz, and pulse width was 200.0–412.5 µs. Interestingly, the commonly used taVNS waveform parameters are remarkably similar to those commonly used in iVNS stimulating the cervical VN.
Summary statistics of stimulation parameters
Stimulation parameter | Median | IQR | ||
---|---|---|---|---|
Overall | Cardiac | Overall | Cardiac | |
Intensity (mA) | 4.0 | 5.66 | 0.85–10.0 | 1.25–13.75 |
Frequency (Hz) | 25.0 | 25.0 | 20.0–25.0 | 20.0–25.0 |
Pulse width (µs) | 250.0 | 200.0 | 200.0–412.5 | 200.0–300.0 |
Summary of the technical parameters of the tVNS studies
Authors (year) | Condition/study | Participants | tVNS device | Stimulation side | Stimulation site | Sham control | Pulse width (µs) | Intensity (mA) | Frequency (Hz) | Duty cycle/time |
---|---|---|---|---|---|---|---|---|---|---|
Keute, et al. (2019) [36] | Visual bistable perception | 34 | DS7, Digitimer | L | Cymba concha | Sham stimulation 25 Hz on ear lobe | 200.0 | 3.0 | 25.0 | 30 s on, 30 s off for 40 min |
Zhao, et al. (2019) [37] | Post-stroke insomnia | 1 | - | L, R | Concha | - | 1,000.0 | 4.0–6.0 | 20.0 | 30 min twice a day for 4 weeks |
Badran, et al. (2018) [38] | Improving oromotor function in newborns | 5 | DS7AH, Digitimer | L | Tragus | - | 500.0 | 0.1 | 25.0 | Max 2 min or less per dose, paired with newborn feeding, stops when newborn stops sucking, up to 30 min a day over 10–22 days |
Badran, et al. (2018) [39] | Neuro-physiologic effects of tVNS | 17 | DS7A, Digitimer | L | Tragus | Sham stimulation 25 Hz on ear lobe | 500.0 | - | 25.0 | Each round consisted of a baseline period of 90 seconds, stimulation period of 60 seconds, and recovery period of 180 seconds |
Colzato, et al. (2018) [40] | Divergent thinking | 80 | NEMOS®, Cerbomed GmbH | L | Concha | Sham stimulation 25 Hz on ear lobe | - | 0.5 | 25.0 | 30 s on, 30 s off for 40 min |
Fischer, et al. (2018) [41] | Conflict-triggered adjustment of cognitive control | 21 | CM02, Cerbomed | L | Cymba concha | Sham stimulation 25 Hz on ear lobe | 200.0–300.0 | 1.3 | 25.0 | Continuously for 36 min |
Jongkees, et al. (2018) [42] | Response selection during sequential action | 40 | CM02, Cerbomed | L | Tragus | Sham stimulation 25 Hz on ear lobe | 200.0–300.0 | 0.5 | 25.0 | 30 s on, 30 s off for 45 min |
Keute, et al. (2018) [43] | Ergic modulation of GABA | 16 | DS7, Digitimer | L | Concha | Sham stimulation 25 Hz on ear lobe | 200.0 | 8.0 | 25.0 | 30 s on, 30 s off for 25 min |
Liu, et al. (2018) [44] | Epilepsy | 17 | TENS-sm device, Suzhou Medical Audio Supplies | L, R | Cymba concha and outer ear canal | - | 200.0 | - | 10.0 | 20 min three times a day for 6 months |
Yakunina, et al. (2018) [45] | Tinnitus | 36 | Custom-made | L | Inner tragus and cymba concha | Sham stimulation 25 Hz on ear lobe | 500.0 | 0.1 | 25.0 | Each location was stimulated in two runs with 30 s of stimulation followed by 30 s of rest; this cycle was repeated five times in a run |
Assenza, et al. (2017) [46] | Epilepsy | 1 | NEMOS®, Cerbomed GmbH | L | External acoustic meatus | Sham stimulation on right ear lobe | - | - | - | 4 h |
Fang, et al. (2017) [47] | Depression | 38 | VN stimulator developed in cooperation with Suzhou Medical Appliance Factory | - | Concha | Sham stimulation 20 Hz delivered to superior scapha | 200.0 | 4.0–6.0 | 20.0 | Continuously for 30 min twice a day, 5 days a week for 4 weeks |
Yu, et al. (2017) [48] | Disorders of consciousness | 1 | - | L, R | Concha | - | 1,000.0 | 4.0–6.0 | 20.0 | 30 min twice a day for 4 weeks |
Bauer, et al. (2016) [49] | Epilepsy | 76 | NEMOS®, Cerbomed GmbH | L | Cymba concha | Active control 1 Hz stimulation | 250.0 | - | 1.0 or 25.0 | 30 s on 30 s off for 4 h |
Burger, et al. (2016) [50] | Fear extinction in health volunteers | 38 | NEMOS®, Cerbomed GmbH | L | Cymba concha | Sham stimulation 25 Hz on ear lobe | - | 0.5 | 25.0 | 30 s on 30 s off |
Cha, et al. (2016) [51] | Sudden-onset vertigo | 1 | ES-420, ITO Co., Ltd. | R | Cymba concha, cavum concha, and outer surface of tragus | - | 200.0 | - | 30.0 | 4 min each site |
Frøkjaer, et al. (2016) [52] | Pain threshold | 18 | NEMOS®, Cerbomed GmbH | L | Concha | Sham stimulation 30 Hz on ear lobe | 250.0 | - | 30.0 | 60 min |
Gaul, et al. (2016) [13] | Chronic cluster headache | 45 | - | R | Neck | - | - | 60.0 | 25.0 | Burst (1 ms on, 40 ms off) for three consecutive 2-minute stimulations, with a 1-minute interval in between |
Grazzi, et al. (2016) [53] | Menstrual related migraine | 51 | gammaCore®, electroCore LLC | L, R | Neck | - | 200.0 | 60.0 | 25.0 | Burst (1 ms on, 50 ms off) for 2 min three times a day |
Lerman, et al. (2016) [54] | Peripheral immune system modulation in healthy humans | 20 | gammaCore®, electroCore LLC | L, R | Neck | Active control 1 Hz stimulation | 200.0 | - | 25.0 | Burst (1 ms on, 40 ms off) for 2 min |
Rong, et al. (2016) [55] | Major depressive disorder | 160 | - | - | Concha | Sham stimulation 20 Hz at superior scapha | 200.0 | 4.0–6.0 | 20.0 | Continuously for 30 min twice a day |
Silberstein, et al. (2016) [15] | Migraine | 59 | gammaCore®, electroCore LLC | R | Neck | Sham device that did not deliver electrical stimulation | - | 60.0 | - | Each treatment consisted of two 2-minute self-administered stimulations delivered 5–10 min apart to the right side of the neck at 3 prespecified times every day |
Silberstein, et al. (2016) [56] | Cluster headache | 150 | gammaCore®, electroCore LLC | R | Neck | Sham device delivering 0.1 Hz biphasic pulse | 200.0 | 60.0 | 25.0 | Burst (1 ms on, 40 ms off) for three consecutive 2 min stimulations 1 min apart |
Trevizol, et al. (2016) [57] | Depression | 12 | Neurodyn II, IBRAMED | L, R | Mastoid process | - | 250.0 | 12.0 | 120.0 | 30 min a day ten times over 2 weeks |
Fang, et al. (2016) [58] | Major depressive disorder | 34 | - | L | Concha | Sham stimulation 20 Hz at superior scapha | 1,000.0 | 4.0–6.0 | 20.0 | 2 times 30 min daily, 5 days a week for 4 weeks |
Frangos, et al. (2015) [25] | Bold fMRI effects of tVNS | 12 | NEMOS®, Cerbomed GmbH | L | Cymba concha | Sham stimulation 25 Hz on ear lobe | 250.0 | 0.3–0.8 | 25.0 | Continuously for 14 min |
Hyvärinen, et al. (2015) [59] | Tinnitus | 15 | Transcutaneous vagus nerve stimulator, Tinnoff Inc. | L | Tragus | Sham stimulation 25 Hz on ear lobe | 500.0 | 0.5 | 25.0 | Continuously for 6 min |
Nesbitt, et al. (2015) [60] | Cluster headache | 19 | gammaCore®, electroCore LLC | L, R | Neck | - | 1,000.0 | - | 25.0 | 2 min per dose, up to three doses twice daily |
Sellaro, et al. (2015) [61] | Post-error slowing | 40 | CM02, Cerbomed GmbH | L | Outer auditory canal | Sham stimulation 25 Hz on ear lobe | 200.0–300.0 | 0.5 | 25.0 | 30 s on and 30 s off for 75 min |
Sellaro, et al. (2015) [62] | Pro-social behavior | 24 | CM02, Cerbomed GmbH | L | Outer auditory canal | Sham stimulation 25 Hz on ear lobe | 200–300 | 0.5 | 25.0 | 30 s on and 30 s off for 26 min |
Altavilla, et al. (2015) [63] | Migraine | 20 | gammaCore®, electroCore LLC | - | Neck | - | - | - | - | Continuously for 90 s |
Barbanti, et al. (2015) [14] | Chronic migraine | 50 | gammaCore®, electroCore LLC | R | Neck | - | - | - | - | 2 times 120 s doses 3 min apart per migraine |
Hasan, et al. (2015) [64] | Schizophrenia | 20 | CM02, Cerbomed GmbH | L | Outer auditory canal | No electrical stimulation delivered | 250.0 | - | 25.0 | 30 s on, 180 s off for up to 3 times 3 h a day |
Jacobs, et al. (2015) [65] | Associative memory in older individuals | 30 | TENStem dental, schwa-medico GmbH | L | External acoustic meatus on inner side of tragus | No electrical stimulation delivered | 200.0 | 5.0 | 8.0 | Twice a day |
Kinfe, et al. (2015) [66] | Cluster-Tic syndrome | 1 | gammaCore®, electroCore LLC | R | Neck | - | 1,000.0 | 12.0–14.0 | 25.0 | Burst for 2 times 90 s doses 15 min apart |
Kinfe, et al. (2015) [67] | Migraine and sleep disturbance | 20 | gammaCore®, electroCore LLC | L, R | Neck | - | 1,000.0 | 0.0–24.0 | 25.0 | Burst for 2 times 2 min twice a day |
Stavrakis, et al. (2015)* [68] | Atrial fibrillation | 40 | Grass® S88, Natus Neurology Inc. | R | Tragus | No electrical stimulation delivered | 1,000.0 | - | 20.0 | Continuously for 60 min following induction of atrial fibrillation |
Steenbergen, et al. (2015) [69] | Efficiency of action cascading processes in healthy humans | 30 | CM02, Cerbomed GmbH | L | Outer auditory canal | Sham stimulation 25 Hz on ear lobe | 200.0–300.0 | 0.5 | 25.0 | 30 s on, 30 s off for 45 min |
Straube, et al. (2015) [23] | Migraine | 46 | NEMOS®, Cerbomed GmbH | L | Concha | Active control 1 Hz sham stimulation | 250.0 | - | 1.0 or 25.0 | 30 s on, 30 s off for 4 h a day for 12 weeks |
Weise, et al. (2015) [70] | Parkinson’s disease | 50 | - | L, R | Tragus | - | 100.0 | 8.0 | 0.5 | - |
Zhi, et al. (2014) [71] | Tinnitus | 32 | TENS-200, Suzhou Medical Supplies Co., Ltd. | - | Cavum concha | - | 1,000.0 | 1.0 | 20.0 | 2 times 20 min daily for 8 weeks |
Aihua, et al. (2014) [72] | Epilepsy | 60 | TENS-200, Hebei Huatuo Pharmaceutical Co., Ltd. | L, R | Outer auditory canal and conchal cavity | Sham stimulation 20 Hz on ear lobe | 200.0 | - | 20.0 | Continuously for 20 min three times a day |
Capone, et al. (2015) [73] | Cortical excitability in healthy volunteers | 10 | Twister, EBM | L | External acoustic meatus at inner side of tragus | Sham stimulation 20 Hz on ear lobe | 300.0 | 8.0 | 20.0 | 30 s on, 270 s off for 1 h |
Clancy, et al. (2014) [74] | SNA in healthy humans | 48 | V-TENS PlusTM, Body Clock Health Care Ltd. | - | Tragus | Disconnected electrodes for sham | 200.0 | 10.0–50.0 | 30.0 | Continuously for 15 min |
Goadsby, et al. (2014) [75] | Acute migraine | 30 | gammaCore®, electroCore LLC | R | Neck | - | - | - | - | 2 times 90 s doses 15 min apart after migraine onset |
Grazzi, et al. (2014) [76] | Migraine | 30 | gammaCore®, electroCore LLC | R | Neck | - | - | - | - | 90 s |
Huang, et al. (2014) [77] | Impaired glucose tolerance | 72 | TENS-200, Hebei Huatuo Pharmaceutical Co., Ltd. | - | Concha | Sham stimulation 20 Hz applied at superior scapha | 1,000.0 | 1.0 | 20.0 | 20 min twice daily for 12 weeks |
Kreuzer, et al. (2014) [78] | Tinnitus | 50 | Phase I: CM02, Cerbomed GmbH; phase II: NEMOS®, Cerbomed GmbH | - | - | - | - | 0.1–10.0 | 25.0 | Phase I: 30 s on, 180 s off for 6 h per day; phase II: 30 s on, 30 s off for 4 h per day |
Laqua, et al. (2014) [79] | Pain threshold in healthy humans | 22 | TNS SM 2 MF, schwa-medico GmbH | L, R | Cavum concha and mastoid area | No electrical stimulation delivered | 200.0 | - | 2.0 and 100.0 | Burst 30 min |
Busch, et al. (2013) [80] | Pain perception in healthy volunteers | 48 | STV02, Cerbomed GmbH | L | Concha at inner side of tragus | No electrical stimulation delivered | 250.0 | 0.3–10.0 | 25.0 | Continuously for 1 h |
He, et al. (2013) [81] | Pediatric epilepsy | 14 | TENS-200 | L, R | Concha | - | - | 0.4–1.0 | 20.0 | 3 times 30 min a day |
Lehtimäki, et al. (2013) [82] | Tinnitus | 10 | Pulse generator, Tinnoff Inc. | L | Tragus | No electrical stimulation delivered | - | 0.8 | 25.0 | 7 times 45/60 min sessions delivered over 10 days |
Kraus, et al. (2013) [83] | Effects of sham-controlled transcutaneous electrical stimulation | 16 | DS7A, Digitimer | L | Group I: anterior wall of ear canal; group II: posterior side of ear canal | Sham stimulation 8 Hz on ear lobe | 20.0 | - | 8.0 | 4 times 30 s on, 60 s off |
Hein, et al. (2013) [9] | Depression | 37 | Study 1: TENS-NET 2000, Auri-Stim Medical Inc.; study 2: TENS-NET 1000, Auri-Stim Medical Inc. | L, R | Outer auditory canal | No electrical stimulation delivered electrodes unplugged | - | 0.13 | 1.5 | Study 1: 1 time 15 min 5 days a week; study 2: 2 times 15 min 5 days a week |
Napadow, et al. (2012) [84] | Chronic pelvic pain | 15 | Cefar® Acus II, Cefar Medical | L | Cymba concha and slope between antihelix and cavum concha | Sham stimulation 30 Hz on ear lobe | 450.0 | - | 30.0 | 0.5 s on, matched to respiration for 30 min |
Stefan, et al. (2012) [1] | Epilepsy | 10 | - | L | Tragus | - | 300.0 | - | 10.0 | 3 times 1 h a day over 9 months |
Schulz-Stübner and Kehl (2011) [85] | Hiccups | 1 | NMS 300, Xavant Technology (Pty) Ltd. | L | Neck | - | - | 6.0 | 1.0 | 30 s |
Dietrich, et al. (2008) [86] | Bold fMRI | 4 | Specially designed MRI-compatible stimulation unit | L | Tragus | - | 250.0 | 4.0–8.0 | 25.0 | 50 s on, 100 s off for 700 s |
Kraus, et al. (2007) [87] | Bold fMRI | 22 | EMP2 Expert, schwa-medico GmbH | L | Tragus | Sham stimulation 8 Hz on ear lobe | 20.0 | - | 8.0 | 30 s on, 120 s off three times over 2 days |
Fallgatter, et al. (2003) [21] | Vagus sensory evoked potentials | 6 | - | - | Tragus and acoustic meatus | - | 100.0 | 8.0 | - | 2 s interstimulus interval |
Johnson, et al. (1991) [88] | Pain threshold and autonomic function | 24 | Microtens 7757 | R | Concha | No electrical stimulation delivered | 500.0 | - | 2.3 | Burst for 15 min |
Andreas (2019)* [89] | Electrocardiogram post atrial fibrillation | - | DUCEST Neurostimulator V, Biegler Medizinelektronik GmbH | - | Triangular fossa | Sham: same location, low-level stimulation | - | 1.0 | 1.0 | 40 min on 20 min off |
Stavrakis (2015)* [68] | Atrial fibrillation cycle length and duration, TNF-α, CRP | Grass® S88, Natus Neurology Inc. | R | Tragus | Placebo: no current, same location | 1,000.0 | - | 20.0 | - | |
Stavrakis, et al. (2020)* [90] | Atrial fibrillation burden | - | ParasymTM clip, Parasym Health Inc. | R | Tragus | Sham: different amplitude (mean 19.9 mA), different location (right ear lobe) | 200.0 | 16.8 | 20.0 | - |
Badran, et al. (2018)* [27] | HRV during stimulation | - | Custom clip | L | Tragus | Sham: at 100 μs pulse width: 6.57 ± 1.83 mA. At 200 μs pulse width: 3.64 ± 1.26 mA. At 500 μs pulse width: 1.97 ± 0.71 mA. Different location (ear lobe) | 100.0 200.0 500.0 | 9.3 5.3 3.0 | 1.0 10.0 25.0 | - |
Antonino (2017)* [91] | cBRS from systolic BP and RR interval HRV (low frequency/high frequency) | - | Ear clip electrodes | L, R | Tragus | Placebo 1 sham: same waveform (mA NR), different location (bilateral ear lobe) | 200.0 | 10.0–50.0 | 30.0 | - |
Bretherton (2019)* [92] | HRV and baroreflex sensitivity | - | Custom TENS electrodes | - | Tragus | Placebo: same location (inner and outer tragus) | 200.0 | 2.0–4.0 | 30.0 | - |
Clancy (2014)* [74] | HRV and sympathetic activity | - | V-TENS PlusTM, Body Clock Health Care Ltd. | - | Tragus | Placebo: no current, same location | 200.0 | 10.0–50.0 | 30.0 | Continuous |
De Couck, et al. (2017)* [93] | ECG with HRV | - | NEMOS®, Cerbomed GmbH | L, R | Cymba concha | Placebo: no current, same location | 250.0 | 0.7 | 25.0 | - |
Tran, et al. (2019)* [94] | LV strain and autonomic tone | - | ParasymTM earclip, Parasym Health Inc. | R | Tragus | Sham: different current (mean 21.8 mA), different location (right ear lobe) | 200.0 | 22.6 | 20.0 | - |
Yu, et al. (2017)* [95] | Myocardial ischemia-reperfusion injury | - | Clip electrodes (S20 stimulator) | R | Tragus | Placebo: no current, same location (right tragus) | 1,000.0 | - | 20.0 | 5 s on/off |
Tobaldini (2019)* [96] | Orthostatic stress, HRV, low frequency/high frequency, systolic arterial BP variance, respiratory rate | - | NEMOS®, Cerbomed GmbH | L | Cymba concha | Placebo: no stimulation, same location | 200.0 | 1.0–6.0 | 25.0 | - |
Fisher (2018)* [97] | Hypertension | - | - | L | Biphasic left cymba concha and beneath antihelix | Placebo: no current, same location | - | 15.0 | 25.0 | 1 s duration (gated to exhalation) |
Stowell (2019)* [98] | Hypertension | - | UROstim device, schwa-medico GmbH | L | Cymba concha | Placebo: no current, same location | 300.0 | - | 2.0 10.0 25.0 100.0 | 1 s on/off |
Zamotrinsky (2001)* [99] | Coronary artery disease | - | Ear clip electrodes | L, R | Tragus | Placebo 1 sham: same waveform (mA), different location (bilateral ear lobe) | 200.0 | 10.0–50.0 | 30.0 | - |
Borges (2019)* [100] | Cardiac vagal activity, HRV | - | NEMOS®, Cerbomed GmbH | L | Cymba concha | Sham: same set stimulation, different strong sensory stimulation (2.76 ± 1.01 mA), different location (ear lobe) | 200.0–300.0 | 12.5 | 25.0 | 30 s on/off |
L: left ear; R: right ear; TNF: tumor necrosis factor; CRP: C-reactive protein; BP: blood pressure; HRV: heart rate variability; LV: left ventricular; GABA: gamma-aminobutyric acid; fMRI: functional MRI; SNA: sympathetic nerve activity; -: no description; *: ardiac related trials
The significant differences in waveform parameters can be explained by the exploratory nature of the tVNS studies. This leads to difficulties in comparisons because studies with similar indications use different parameters chosen by the researchers.
Whereas differences in pulse width and frequency can be explained as choices of researchers, the source of large variation in stimulation intensity is not as trivial. Differences in details such as material and design of electrodes, area, and stimulation polarity must be considered. This thesis is supported by Poulsen et al. [101] who found that contact area and electrode geometry have the potential to influence a target engagement of underlying nerves.
An overview of the number of stimulation parameters used in cardiac trials and comparison them to the overall statistics is presented in Table 1. For further details, the reader is referred to Table 2.
All data from Table 2 are illustrated in a whisker boxplot, as shown in Figure 2. Shown are the IQR, minima, maxima, and medians of the stimulation waveform parameters, including the extreme cases.
Regarding the location of stimulation, the review by Yap et al. [31] and Verma et al. [35] shows that 50% of the studies target the left ear, only about 20% target the right ear, and 20% target both ears. About 10% of the studies do not provide any details. The most identified areas of stimulation are the neck in tcVNS and the tragus or cymba concha in taVNS.
The visualization of stimulation parameters is not a reason to apply these parameters in future studies but is intended to provide an overview of recently used parameters.
To summarize, a closer look into the mechanism of the VN stimulation and its effects on brain stimulation is necessary. The results would lead to a wider range of treatment options, e.g., in cardiovascular diseases, adjuvant cancer, or gastrointestinal issues.
In the end, universally valid parameters would help achieve constant results and reduce potential side effects. Only could the requirements for FDA approval be fulfilled.
Current clinical uses of tVNS
Depression
iVNS and tVNS already play an influential role in therapy-resistant depression, although the real mechanism behind the therapeutic anti-depressive effects is still unknown. In 2013, a placebo-controlled study by Hein et al. [9] showed that 2 weeks of tVNS treatment decreased depression severity.
This finding was replicated later, in a non-randomized study with a larger patient sample, but only a third of the sample responded to tVNS treatment [55].
A study by Kraus et al. [87], published in 2007, has thematized the acute brain activation of healthy subjects treated with tVNS. Thereafter, fMRI revealed the hypoactivation of the limbic brain areas, including hippocampus, amygdala, middle and superior temporal gyrus, and parahippocampal gyrus. Hyperactivation was visible in insula cortex [102–104], thalamus, and precentral gyrus. This is in line with previous studies [83, 87]. They confirm the feasibility and positive effects of tVNS in the left auditory canal of healthy subjects. Similarities were found in the activation pattern of the brain during iVNS and tVNS. These revelations are key, due to their connection to the NTS, which receives the greatest input of vagal afferents [87]. To understand the importance of the NTS in VNS treatment, one must think of the NTS as a relay between sensory information arriving via vagal afferents and the brain along an autonomic feedback loop. Direct projections transmit information to the reticular formation of medulla, while ascending projections conduct to amygdala, hypothalamus, thalamus, insula, and orbitofrontal cortex. Furthermore, mood regulation and anxiety affected by limbic regions are controlled by locus coeruleus and parabrachial nucleus [105].
Due to this, the suppression of the hyperactive limbic brain areas can be hypothesized to be caused by the hypoactivation of amygdala [106], which in turn may decrease the severity of depression.
This is consistent with the results of subjects with depression treated with VNS [105, 107, 108].
Neuroimaging studies of patients with depression demonstrated that tVNS influences functional brain connectivity in the default mode network [58] and the activation of the insula [47], which is a central area in relation to the pathophysiology of depression [109]. A reduction in depression severity can be observed [58] and it also correlated to results seen in the therapeutic effects of transcranial magnetic stimulation [110]. Furthermore, a decrease in functional connectivity between hypothalamus and rostral anterior cingulate cortex (rACC) [111] was seen. In addition, an increase in functional connectivity between the left nucleus accumbens and the bilateral rACC was noted during tVNS treatment [112].
The “bottom-up” mechanism is a hypothesis by Shiozawa et al. [113] based on the neurobiology of the VN and its effects on neuronal activity. Its principle is the activation of the central nervous system by electrical stimulation of peripheral nerves, especially the VN.
This mechanism contradicts the “top-down” mechanism of therapy strategies (e.g., transcranial magnetic stimulation). This strategy uses a stimulus applied to central brain structures to generate an impulse to peripheral sites.
Tinnitus
About 10–15% of the general population is affected by a dysfunction of the ear, where people perceive sounds in absence of actual external sounds [114]. Based on recent studies, the assumption that chronic tinnitus is linked to the auditory system was made by Lehtimäki et al. in 2013 [82]. Due to this, abnormal neuronal behavior has been observed [82, 115].
Hearing impairment and related tinnitus often accompany dysfunction of the balance organs (vestibular system) [102]. Some vestibular disorders associated with it include Ménière’s disease and secondary endolymphatic hydrops (resulting from abnormal amounts of a fluid called endolymph collecting in the inner ear) and perilymph fistula (a tear or defect in one or both of the thin membranes between middle and inner ear) [116–118].
In rat studies, VNS stimulation combined with sound therapy has generated positive effects on tinnitus. Based on these realizations, a pilot study was performed [115]. The intention was to figure out whether tVNS can show similar effects on patients with chronic tinnitus.
At the end of the study, all participants reported less severity of tinnitus and an improved mood. This is consistent with several other studies investigating the efficacy of tVNS in the treatment of tinnitus. tVNS has been shown to be effective and safe [45, 119, 120]. Very good results have been obtained in modulating beta and gamma band activity associated with tinnitus [59], in the feasibility and safety of tVNS in conjunction with notched music therapy [121], and in the investigation of effects of tVNS on ANS imbalance, which is common in tinnitus patients [122].
In addition, magnetoencephalography scans demonstrated that tVNS treatment can influence and modulate the auditory system [82].
However, another pilot study, using tVNS, but not combined with sound therapy, did not show the same clinical effects [78]. It can be assumed that an improvement in tinnitus is seen, through suppression of auditory, limbic, and other brain regions [45].
As a result, the idea emerged that tVNS together with a sound therapy can improve neuronal plasticity. In addition, a reduction in the activity of the gamma band in the left auditory cortex is noticed as well as phase coherence between the cortex [123].
Epilepsy
VNS has also been investigated for its treatment in drug-resistant epilepsy, a neurological disorder, that causes seizure attacks. Today, there a about 50 million people worldwide affected by epilepsy [124] and up to 30% are diagnosed as drug resistant [125]. This relatively high number of cases led to research for alternative treatment options.
Already in 1998, studies have demonstrated that iVNS has positive effects on seizures. A new alternative, the non-pharmacological treatment option, has been established [126]. Due to great results in iVNS, pilot studies were devised to investigate whether tVNS can evoke similar anti-convulsive effects. After 9 months, five out of seven patients showed lower seizure frequencies [1]. This reduction could also be confirmed over a larger sample and a longer period (12 months) [72].
He et al. [81] demonstrated comparable results in a pediatric study. Another study, a randomized clinical trial observed 47 patients over 24 weeks. In the end, 16% were seizure free and about 38% had a decrease in seizure frequency [127].
Recent meta-analysis provided the evidence for tVNS on epilepsy [128–130]. Until now the exact mechanism of tVNS in epilepsy is not well-understood. But there are several ideas, that afferent projections of the ABVN, leading to the NTS may be responsible for the anti-epileptic effect. However, the neuronal networks projecting downstream are not fully understood [131]. Nevertheless, the NTS could be linked to the locus coeruleus and raphe nuclei, which are brain areas associated with seizures [132].
A suggestion as to why tVNS can affect seizure frequency was made by Krahl et al. [132] and Marrosu et al. [133]. They argued that it is based on the activation of inhibitory structures in the brain, due to an increase of free GABA [134] or with an increase in norepinephrine [135].
In 1990, studies on rats already indicated that recruitment of C-fibers has positive effects on epilepsy. The activation of C-fibers of the VN, and in that way, a mediation of GABA and glycine levels promotes the suppression of seizures [136]. This in turn is consistent with the statements by Fu et al. [137].
Migraine
There are studies looking towards reducing the frequency of migraine attacks and improving the quality of life.
The main selected treatment option in all studies was the gammaCoreTM device, a tcVNS, hand-held against the neck to stimulate CBVN. Total stimulation time was about 90 s with a frequency of 25 Hz via steel skin surface electrodes, placed on the neck. Excitingly, fewer migraine attacks and a reduction in severity and disability of attacks were observed [14, 16, 67, 75].
Since the trials with tcVNS were successful, the question is whether taVNS can achieve similar results in the treatment of migraine.
In 2015, Straube et al. [23] used the NEMOS® taVNS device to perform the studies. In total, 46 patients were tested with the NEMOS® device, the electrodes were applied to the tragus and worked with a frequency of 25 Hz for 4 h per day. The tests lasted 3 months. As an active control group, they used a stimulation frequency of 1 Hz. Interestingly, in the test with 1 Hz, they achieved greater results. However, in both groups, they could observe a reduction in the number of migraine attacks [23].
As with the other current clinical applications, e.g., in chronic epilepsy, the exact mechanism of tVNS is still unknown. All information is founded on hypotheses derived from experience in studies.
One theory’s foundation is based on the fact that activation of the thalamus plays a key role in information processing and regulation of cortical activity. The stimulation of vagal afferents has led to the activation of the NTS, which relates to the thalamus.
fMRI studies of patients with migraine have shown a decrease in thalamocortical activity. According to this, activation of the thalamus via VNS could be an important factor in reducing the severity of headaches [135–137].
After considering the above clinical applications of tVNS, it is evident that the exact mechanism of tVNS is not well understood. Studies have proven that tVNS has potential as a treatment option by showing significant effects in treating symptoms. To achieve tVNS becoming more than just an alternative option, but a first-line treatment method, further investigations must be made.
New clinical applications of tVNS
Modulation of inflammation
An exciting new application of VNS is an anti-inflammatory treatment. This idea is based on the fact that the inflammation process is regulated through humoral and neuronal reflex pathways [138, 139]. It is specifically caused by a disbalance of metabolic and immune functions. Furthermore, disease progression may result from imbalances between pro-inflammatory and anti-inflammatory cytokines [140]. This is the reason for the progression of numerous chronic diseases such as cardiovascular diseases, arthritis, Alzheimer’s disease, and diabetes mellitus.
An untreated inflammatory event can lead to chronic inflammation that is unable to reestablish cell and tissue homeostasis due to an excessive immune/tissue response, as demonstrated in Figure 3. As a result, tumor-promoting inflammation may be a consequence and accelerate tumor progression.
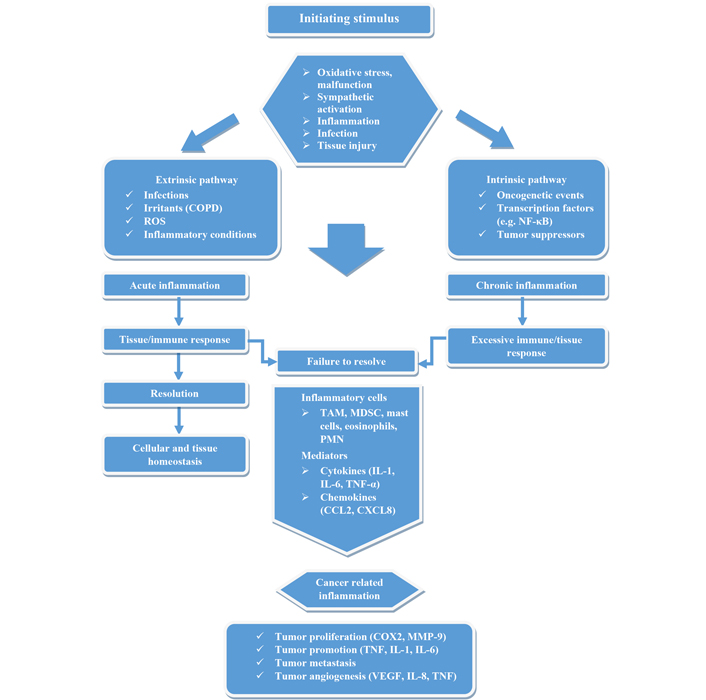
Visualization of the development of cancer-related inflammation. Based on initial stimuli (intrinsic, extrinsic), acute or chronic inflammation enters. If the immune/tissue response is not sufficient and homeostasis does not develop, then chronic inflammation favoring cancer may occur due to inflammatory mediators. NF-κB: nuclear factor-kappa B; COPD: chronic obstructive pulmonary disease; ROS: reactive oxygen species; TAM: tumor-associated macrophages; MDSC: myeloid-derived suppressor cells; PMN: polymorphonuclear neutrophils; IL: interleukin; CCL2: chemokine (C-C motif) ligand 2; CXCL8: chemokine (C-X-C motif) ligand 8; COX2: cyclooxygenase-2; VEGF: vascular endothelial growth factor; MMP: matrix metalloproteinase
These findings supply convincing evidence that the stimulation of the VN plays a key role in defense against infections and inflammations.
Whereas the sympathetic nervous system (SNS) may have both anti-inflammatory and pro-inflammatory effects, the parasympathetic system has only anti-inflammatory effects. This is provided in the inverse relationship between parasympathetic markers of HRV and inflammatory markers [141].
In general, the VN can be associated with three reflex pathways which show clear positive anti-inflammatory effects:
There is the anti-inflammatory vago-vagal reflex, more precisely the cholinergic anti-inflammatory pathway [142, 143]. The infection causes the activation of the VN fibers and leads to a release of acetylcholine at the synapses of efferent fibers. Acetylcholine can bind onto the surface of macrophages and suppress the production of cytokines [144].
Another pathway is the anti-inflammatory hypothalamic-pituitary-adrenal axis. It was noted that injuries and infections activate via pro-inflammatory cytokines and conduct information to the NTS. Activation of somatotopic area of the NTS leads to an excitation cascade via the hypothalamus and hypophysis. Lastly, there is a release of glucocorticoids by the adrenal glands. Glucocorticoids play a vital role in the decrease of peripheral inflammation. This is, for instance, consistent with some research articles that have investigated the direct influence of glucocorticoids on the integrity of blood-brain barrier in neuroinflammatory conditions. The results suggest that glucocorticoids are effective in stabilizing the blood-brain barrier and thus positively influence neuroinflammatory diseases such as multiple sclerosis, encephalitis, meningitis, acute ischemic stroke, and brain tumors [145–147].
The third pathway is the splenic sympathetic anti-inflammatory pathway. Here, also efferent VN fibers are key in releasing norepinephrine by the endings of adrenergic sympathetic nerves in the spleen [144].
Norepinephrine is decisive in the release of acetylcholine by lymphocytes. The importance of acetylcholine in inhibiting macrophages is described above.
Regarding these reflex pathways, it can be assumed that the activity of VN is important in maintaining homeostasis by limiting inflammatory responses. This leads to the questions: How VNS can positively affect this homeostasis and avoid immunosuppression and whether VNS can show benefits in the treatment of chronic diseases correlated with inflammation.
A clinical trial investigating the suppression of atrial fibrillation by Stavrakis et al. [68] already showed that the modulation of VN via taVNS reduces the production of pro-inflammatory cytokines (TNF-α and CRP). TNF-α is present in the acute inflammatory response and is known as one of the most conditional mediators in inflamed tissue [148]. In addition, active group subjects treated for myocardial infection have reported a significant reduction of all measured cytokine levels [TNF-α, IL-6, IL-1β, and high mobility group-box 1 protein (HMGB1)] [95]. In contrast, a trial performed by Salama et al. [149] couldn’t show a significant change in TNF-α levels, but in lower levels of CRP and IL-6, compared to the control group. CRP, an acute phase protein, is released by the liver in response to higher levels of IL-6.
In rats, positive effects on rheumatoid arthritis during VNS treatment have been seen [150].
In humans, the potential of taVNS is related to chronic inflammatory conditions and has been reviewed in trials thematizing, e.g., postoperative ileus [143, 151].
Considering these studies, there is unambiguous evidence that VNS may have a positive effect on maintaining homeostasis by limiting pro-inflammatory markers. This is a reason to widen VNS treatment in chronic diseases.
In particular, the implication of lowered circulating cytokine levels on chronic diseases needs to be quantified in further investigations, to achieve greater clinical relevance.
Can the long-time survival in cancer therapy be positively affected by VNS, in terms of inflammation modulation?
Cancer is the second leading cause of mortality worldwide. Prostate cancer in men and breast cancer in women are the most common types of cancer are named [152]. Although cancer is known as a complex disease, including hundreds of different types, there are several characteristics which cancer types have in common. The most important is resisting cell death (apoptosis), the induction of angiogenesis, performing invasion and migration, the evasion of tumor growth suppressors, or enhancing cell proliferation.
Recent studies have now shown that there are three biological factors which have a great influence on the tumorigenesis and its progression: oxidative stress [153, 154], inflammation [154–156], and excessive sympathetic activity [157].
There are some cancer studies (non-small cell lung cancer, breast cancer) that investigate the importance of ANS homeostasis in tumor progression. They all have in common that the stimulation of the parasympathetic nervous system reduces tumor growth, as evidenced, for example, by downregulation of immune checkpoint molecules [programmed death-1 (PD-1)] [158], and that increased SNS activity has tumor-promoting effects. These findings suggest that tumor autonomic innervation plays a significant role in regulating tumor growth and progression [158–160]. From the studies made by Reijmen et al. [160], it can deduced that tVNS treatment has improved local antitumor immunity both as monotherapy and in combination with radiotherapy. For example, tVNS treatment enhanced the cytotoxic profile in patients with lung tumor [160].
Studies that have investigated the association between VN activity and the predicted prognosis in cancer lead to the assumption that there is a correlation between HRV and survival time [161–163].
Chiang et al. [163] have shown a significant correlation between survival time and HRV in patients with terminal hepatocellular carcinoma (r = 0.44; P = 0.01) [163]. In addition, the trial conducted by Hoffmann et al. [161] on patients with carcinoid heart disease demonstrated that there is a higher mortality for patients with a lower HRV. After viewing the studies of Fadul et al. [162], a significant association between survival time and standard deviation of normal-to-normal beat interval (SDNN, P > 0.05) is predictable.
Furthermore, Giese-Davis et al. [164] analyzed and evaluated information on women with recurrent and metastatic breast cancer. The results showed that a significantly longer survival time correlated with a high frequency-HRV. Nevertheless, these results were only valid for those without visceral metastases [164].
Regarding studies dealing with SDNN in patients with advanced cancer, there is one to highlight. De Couck et al. [165] are the only ones who described that the relationship between SDNN and survival is mediated by reduced levels of CRP.
This study discusses that there is an inverse correlation between HRV and CRP that may reduce the risk of death in cancer patients. Interestingly, HRV did not correlate with CRP in patients who lived up to one month after diagnosis, but in patients who survived longer, an inverse relationship was observed between HRV and CRP. These findings are in line with considerations made by Gidron et al. [166]. It is notable that VNS may have the potential to modulate cancer progression, especially advanced and/or severe cancer, through neuroimmunomodulation.
In consideration of the impact of VN activity in cancer prognosis, it must be paid attention to vagotomy, the surgical dissection of fibers of the VN. Its purpose is to reduce acid secretion and to control ulcerations in the intestinal tract [167].
After treating ulcer patients with a surgical vagotomy, several follow-up studies have shown an increased risk of developing cancer, like colorectal cancer [168], prostate cancer [169], and pulmonary carcinoma [170].
From this, it can be derived that a relationship between a compromised VN activity and a worse prognosis in cancer is likely. Due to this, it is questioned whether VNS, especially tVNS has therapeutic effects in cancer and whether a clinical treatment concept can be developed from it. As mentioned above, the three biological factors: Inflammation, oxidative stress, and sympathetic activation may have a distinguished role in tumor prognosis. We already discussed the clinical use of VNS in issues caused by inflammation. This is shown, e.g., in a clinical trial, using a tVNS-device to reduce inflammatory markers (IL-1β, IL-8, TNF-α) [54].
The above study shows that there are arguments in favor of treating patients with advanced cancer and/or metastases with tVNS. tVNS can significantly improve the survival rate of patients with terminal cancer, but it does not replace treatments that shrink the tumor, such as chemotherapy or radiation.
Besides, the VN has a mainly homeostatic role in our system. So, it is possible that VNS may have positive effects in slowing down tumorigenesis, even though the primary transmitter of the VN is at the local level of tumor-promoting effects.
Similar dual effects are also observed in other forms of immune defense, e.g., corticotropic releasing hormone, norepinephrine, and other corticosteroids [171].
Nevertheless, it is possible that stimulation of the VN by a taVNS device has systemic rather than local effect. This is due to properties of the ABVN, which consists of approximately 80% afferent fibers leading to the NTS.
On the basis of the present studies, it can be said that the VNS, for example, through the tVNS, is able to improve cancer prognosis and survival indirectly through its immunomodulatory and antidepressant effects.
Electrical stimulation of the VN may trigger the production and activation of antitumor immune cells and immune responses. Therefore, the use of electrical stimulation to modulate the immune system in various ways may be a promising approach for the treatment of cancer [172]. In addition, a recent animal study showed that noninvasive transcutaneous stimulation of the VN improved myocardial performance in doxorubicin (DOX)-induced cardiotoxicity [173]. DOX, one of the most effective antitumor antibiotics in the anthracycline group, is widely used to treat various cancers such as leukemias, lymphomas, and solid tumors [174, 175]. However, the clinical use of DOX is limited by its severe cumulative dose-dependent cardiotoxicity, which can lead to irreversible cardiomyopathy and even congestive HF [175].
tVNS has an inhibitory effect on the cancer itself, and at the same time, it is thought to inhibit chemotherapy-induced cardiotoxicity. Specifically, tVNS has the potential to noninvasively suppress the development of HF associated with cancer treatment.
However, more studies are needed to better establish the role of tVNS in cancer prognosis. More data on “treatment of inflammation-related diseases” needs to be collected and analyzed with respect to adjuvant cancer treatment. To unlock the full potential of tVNS and understand how it can be used to improve cancer prognosis, studies need to be more consistent in terms of methodological aspects to produce more reliable results and overcome shortcomings. Only then can tVNS be considered an effective adjuvant therapy in cancer treatment, both in terms of survival and quality of life.
Cardiovascular events
In modern society, HF is taking on an increasingly important role. Nowadays, due to advanced medical health care, an aging population is clear. Even so, unhealthy lifestyles lead to increased health problems. HF isn’t usually caused by a single event and is often noted as a consequence of other sicknesses such as type 2 diabetes or arteriosclerosis. Treatment-resistant hypertension (TRH) is one of the main risks for cardiovascular events.
After investigating the pathophysiological mechanisms of TRH, an elevated aldosterone level, increased SNA and a sodium retention was noted [176–178]. This is in line with research of Malpas et. al. [179], who consider an increased SNA a key factor for cardiovascular diseases. Further studies confirm the thesis that a cardiovascular system regulated by the central autonomic network is separated into parasympathetic and sympathetic functions. Information from the periphery reaches the brain via afferent nerves and sends efferent signals back to the target organs, such as the cardiovascular system.
Recent guidelines of the European Society of Cardiology (ESC), define TRH as a failure to treat a BP below 140/90 mmHg. Although patients are treated with three antihypertensive medications and at least one diuretic, BP control is not achieved [180].
With regard to TRH and chronic HF, a correlation is seen in the “ESC guidelines for the diagnosis and treatment of acute and chronic heart failure” (McDonagh et al., 2021) [181]. Hypertension is considered a causative factor for the development of HF, especially HFpEF, renal failure, or stroke. Analysis of regional SNA (norepinephrine spillover or microneurography) in human hypertension supports this relationship by showing activation of sympathetic outflows to the heart, kidney, and skeletal muscle vessels [182–184]. Meanwhile, some clinical studies have shown that treatment of patients with HFpEF and HF with reduced ejection fraction (HFrEF) with iVNS is feasible regardless of the site of stimulation. The results suggest that they are well tolerated and feasible in patients with HFrEF. Moreover, improvements in efficacy parameters and in cardiac function and HF symptoms were observed during 12-month follow-up [185, 186].
Furthermore, HFpEF has been studied in relation to neuromodulation with low-level tragus stimulation (LLTS). Patients treated with LLTS showed improvements in inflammatory cytokines, longitudinal global burden, and quality of life [187, 188]; besides, inflammatory cytokines, macrophage infiltration, and fibrosis decreased. Compared to the control group, life expectancy increased [189].
Until now, the therapy recommendation according to ESC guidelines (2021) is an angiotensin receptor/neprilysin inhibitor and a sodium-glucose co-transporter 2 inhibitor (SGLT2i) for HFrEF. No guideline-directed treatment could decrease symptoms and mortality in HFpEF patients [181].
However, BP control is important in the course of HFpEF treatment.
Several different studies investigated HFpEF patients with high BP, who were treated medicinally against hypertension. It was possible to observe the improved prognosis of HFpEF during angiotensin receptor/neprilysin inhibitor treatment [190]. SGLT2i (empagliflozin, dapagliflozin) also improved the outcome of HFpEF patients [191, 192].
A well-established model for the regulation of arterial BP is the “renal-body fluid feedback mechanism.” The model focuses on the idea that BP is determined by the renal function curve. The renal-body fluid feedback is dependent on pressure natriuresis, which enables the ability of kidney to adjust to changes in arterial pressure by altering the renal excretion of water and salt [193–195].
Furthermore, it is often considered that acute changes of BP can be compensated by cardiac output or increased peripheral resistance, while changes in urine output play a more important role in keeping BP at a certain level [196].
According to investigations by Guyton and colleagues [193–195], the pressure natriuresis relationship is key for a stable BP and an imbalance has a critical influence on the development of hypertension.
To understand the significance of SNA, it is important to recognize that the kidney is innervated by both afferent and efferent renal nerves. These nerves terminate at arterioles, the juxtaglomerular apparatus, and the proximal tubule. This is the reason how changes in renal SNA influence renal blood flow, glomeration filtration rate, and urinary sodium and water excretion [197].
After analyzing the influence of renal SNA in terms of fluid balance, it seems useful to investigate the potential of neuromodulation with regard to enhancing the management of HFpEF.
To support this thought process, a closer view of sympathetic denervation is necessary. Renal denervation is a catheter-based procedure to ablate renal sympathetic nerves with radiofrequency. Several studies have drawn on this procedure and distinct reductions in the magnitude of hypertension were regarded [198–201].
Another animal trial investigated long-term, low-dose infusions of norepinephrine into the renal artery of dogs. This has led to water and sodium retention and is due to increased arterial pressure [202, 203].
These studies confirm that SNA may have effects on BP control. It seems highly likely that an increased sympathetic excitation is the cause. If inhibiting the SNS improves HFpEF and hypertension positively, a treatment option by the parasympathetic system, more precisely by activating the VN should lead to further investigation.
To date, only a few animal studies address hypertension and VNS [204–206]. In these studies, an association between VNS treatment and increased HRV and slower progression of chronic hypertension without altering fibrosis in hypertensive rats.
In Dahl salt-sensitive rats, LLTS reduced inflammatory cytokines, macrophage infiltration, and myocardial fibrosis, and improved cardiac function [189]. tVNS, for just 1 h, significantly suppressed atrial fibrillation and decreased systemic inflammatory cytokines [68, 90]. tVNS was shown to suppress atrial fibrillation inducibility by inhibiting the neural activity of major ganglionated plexi within the intrinsic cardiac ANS [207]. tVNS ameliorated diastolic dysfunction in an animal model of HFpEF [188] and acutely improved LV strain in humans [94]. In the 52 patients with HFpEF, global longitudinal LV strain and TNF-α levels at 3 months were significantly improved in the active tVNS group compared to the sham group. The reduction in TNF-α levels correlated with global longitudinal strain improvement [187].
Interestingly, there is only one case report demonstrating the positive effects of tVNS on renal congestion in HFpEF. This case report is about a 77-year-old patient with HFpEF, who showed symptoms of chronic kidney disease, hypertension, type 2 diabetes, and atrial fibrillation. SGLT2i [luseogliflozin (2.5 mg/day)] was added to the current medications. After 3 months, he successfully lost body weight, without deterioration of the kidney function. Nevertheless, a month later, he refused this medication and the symptoms worsened again. In the next step, a tVNS device (frequency of 20 Hz, stimulation amplitude of 1 mA below the level that caused mild discomfort, and a pulse width of 200 ms) was amplified. Just one hour later, the patient’s symptoms ameliorated and the intrarenal venous flow changed to a discontinuous pattern with a systolic interruption [208].
Beyond this, the recent studies by Nagai et al. [208] suggest that tVNS-mediated parasympathetic nerve activation has beneficial effects on BP control by inhibiting sympathetic activation. This positively affects HFpEF and hypertension [209] and causes a reduction of afterload in acute HF patients leads [210].
In addition to hypertension, myocardial ischemia is also considered a major cause of HF. Thus, a research study in rats exposed to 30 min of ischemia and 4 h of reperfusion demonstrated a cardioprotective effect of VNS. IL-17A has been shown to play an important role in ischemia/reperfusion (I/R) injury of the myocardium. The experiment in the rats suggests a direct relationship between VNS and IL-17A. During reperfusion, VNS was able to decrease infarct size. In addition, VNS significantly reduced the release of inflammatory mediators and oxidative stress and prevented cardiomyocyte apoptosis, suggesting that VNS has a cardioprotective effect but is also related to inhibition of the expression of ischemia-promoting IL-17A [211, 212].
Another important aspect of the cardioprotective role of VNS in myocardial ischemia is the downregulation of MMP-9 and transforming growth factor (TGF)-β1. In this context, LLTS is thought to significantly improve LV remodeling after myocardial infarction.
In an animal study of dogs with ligation-induced myocardial infarction, stimulation by LLTS appears to confirm this. Here, follow-up studies show an improvement in ejection fraction and a reduction in interstitial fibrosis and collagen degradation compared with the control group. In addition, elevated MMP-9 and TGF-β1 levels in LV tissue are reduced in dogs with LLTS therapy [213]. These results are consistent with another animal study [214].
In conclusion, the first interesting findings on the use of tVNS to improve renal congestion in patients with HFpEF were presented. Unlike renal denervation, it is a reversible procedure, and unlike drugs, fewer side effects are expected. To be more certain about the potential of tVNS in HFpEF and hypertension, however, further studies in this direction need to be performed and the samples quantified.
Improving auditory processing
Another potential role could be to improve auditory processing. As discussed in the previous section, “current clinical uses”, VNS combined with tone therapy has been shown to be useful, safe, and effective. A number of preclinical and small pilot human studies support this assumption of combining VNS with tones to improve auditory processing, e.g., in patients with tinnitus [45, 119, 120]. Data from an animal study show that VNS paired with specific tones improved tinnitus perception in a rat model and reversed the abnormal plasticity of the primary auditory cortex associated with tinnitus [115]. Not only do animal studies indicate the feasibility of tVNS paired with tone therapy, but pilot clinical studies are investigating the feasibility and safety of VNS paired with tones in patients with moderate to severe chronic tinnitus [215]. These studies found that about 50% of patients in the group that were paired with VNS tones had clinically meaningful improvements in their tinnitus, compared with 28% in the control group after 6 weeks of treatment.
In light of these significant results, it should be imperative that studies on this topic be forced and pushed forward.
Conclusions
The studies discussed here give convincing evidence that tVNS is a safe and effective method of neuromodulation. Specifically, modulating the ANS by inhibiting the detrimental sympatho-dominant processes plays an important role in tVNS.
To determine the most effective stimulation parameters, Yokota et al. [216] examined how tVNS alters ANS activity by comparing the effects of different tVNS frequencies and intensities. In the 35 healthy adult participants, stimulation at an intensity of 3 mA at all frequencies of 100 Hz, 25 Hz, 10 Hz, 1 Hz, and 0 Hz resulted in a significant reduction in heart rate, with stimulation at 100 Hz having the strongest effect. In addition, frequencies of 100 Hz were found to require an intensity of 3 mA to produce the most pronounced effect. Furthermore, participants with higher baseline sympathetic activity showed a stronger parasympathetic response during stimulation, and there may be gender differences in autonomic responses to the application of tVNS. Women responded more strongly to tVNS than men. Women responded more strongly to tVNS than men [216].
In this regard, the frequency of 100 Hz and the intensity of 3 mA might be the most effective setting to elicit increased activity of the VN. However, in the clinical study of cardiovascular diseases, especially HF and atrial fibrillation, the setting of 20 Hz was inferior to the frequency of 100 Hz and the intensity of 1–2 mA [68, 210]. It has been shown that higher intensity is associated with the perception of discomfort in patients with HF [217]. Discomfort thresholds may differ in healthy adults and in patients with heart disease. Further studies are considered necessary to establish parameters for tVNS.
Although there is still too little data on the mechanism of action and no clear parameters of use, this technique has been shown to be effective for conditions such as migraine, tinnitus, and depression. Particularly, the reduction or suppression of excessive inflammation is currently the most promising thread for the establishment of tVNS treatment as an adjuvant therapy option. The improvement of peripheral blood flow due to the reduced sympathetic activity reinforcing should also be mentioned. Most importantly, tVNS has been shown to be safe and convenient, and has few minor side effects.
More new insights into the importance of the role of the VN in ANS are progressively expanding potential applications. By implementing the latest findings in the treatment of diseases related to the ANS, tVNS-mediated neuromodulation may represent an adjuvant therapy to reduce inflammation in advanced cancer and lower hypertension. Nonetheless, tVNS is far from mature but is gaining importance as a new, effective treatment modality by exploiting the body’s own protective mechanisms.
Knowing that the SNS dominates in chronic inflammation, modulation of the antagonism and the parasympathetic nervous system could lead to an expansion of treatment options in inflammatory diseases. This could reduce patient morbidity and thus improve quality of life and could also be of great interest in the treatment of autoimmune diseases associated with an imbalance of the immune system. This applies to diseases such as rheumatoid arthritis, Crohn’s disease, multiple sclerosis, and diabetes. Further systematic studies in this direction are needed to overcome the current limitations in terms of the effects of stimulation parameters and brain activation. tVNS could be the forerunner of an entirely new therapeutic era in which patients are no longer treated with drugs alone but use electrotherapeutics as adjuvant treatments. This new method of modulating the ANS could lead to a new understanding of how the body is affected by the VN.
Abbreviations
ABVN: |
auricular branch of the vagus nerve |
ANS: |
autonomic nervous system |
BP: |
blood pressure |
CBVN: |
cervical branch of the vagus nerve |
CRP: |
C-reactive protein |
DOX: |
doxorubicin |
ESC: |
European Society of Cardiology |
FDA: |
Food and Drug Administration |
fMRI: |
functional magnetic resonance imaging |
GABA: |
gamma-aminobutyric acid |
HF: |
heart failure |
HFpEF: |
heart failure with preserved ejection fraction |
HFrEF: |
heart failure with reduced ejection fraction |
HRV: |
heart rate variability |
IL: |
interleukin |
IQR: |
interquartile range |
iVNS: |
invasive vagus nerve stimulation |
LLTS: |
low-level tragus stimulation |
LV: |
left ventricular |
MMP: |
matrix metalloproteinase |
NTS: |
nucleus tractus solitarii |
SDNN: |
standard deviation of normal-to-normal beat interval |
SGLT2i: |
sodium-glucose co-transporter 2 inhibitor |
SNA: |
sympathetic nerve activity |
SNS: |
sympathetic nervous system |
taVNS: |
transcutaneous auricular vagus nerve stimulation |
tcVNS: |
transcutaneous cervical vagus nerve stimulation |
TNF: |
tumor necrosis factor |
TRH: |
treatment-resistant hypertension |
tVNS: |
transcutaneous vagus nerve stimulation |
VN: |
vagus nerve |
VNS: |
vagus nerve stimulation |
Declarations
Author contributions
NF: Conceptualization, Investigation, Writing—original draft, Writing—review & editing. MN: Validation, Writing—review & editing. CYF: Conceptualization, Investigation, Validation, Writing—review & editing, Supervision. All authors have read and approved the submitted version.
Conflicts of interests
The authors declare that they have no conflicts of interest.
Ethical approval
Not applicable.
Consent to participate
Not applicable.
Consent to publication
Not applicable.
Availability of data and materials
Not applicable.
Funding
This work was funded by grant Deutsche Forschungsgemeinschaft [FO 315/5-1 to CYF]. The funders had no role in study design, data collection and analysis, decision to publish, or preparation of the manuscript.
Copyright
© The Author(s) 2023.