Abstract
This review focuses on the current advances in the field of therapeutic targets and treatments for stroke. Stroke is a major health problem worldwide, with significant impacts on morbidity and mortality, and a considerable burden on the medical and socio-economic systems. This review provides a comprehensive overview of the current state of knowledge on acute treatments and therapeutic targets. Current stroke treatments like recanalization therapies focus mainly on restoring blood flow to the brain, reducing cell death, and preventing further damage, but have limitations in terms of efficacy and long-term outcomes. Besides acute treatments (mobile stroke units, telerehabilitation) and acute therapeutic targets, the review focuses on longer-term therapeutic targets, such as neuroprotection and neuroregeneration. Neuroprotective strategies target the mechanisms underlying energy failure, cellular acidosis, mitochondrial dysfunction, endoplasmic reticulum stress, excitotoxicity, calcium channels dysregulation, oxidative stress, neuroinflammation, blood-brain barrier disruption, apoptosis, and ischemia-reperfusion injury. Neuroregenerative approaches include stem cell therapy, gene therapy, growth factors, and rehabilitation techniques that promote the rewiring of neuronal circuits in the brain. Non-pharmacological treatments like neurostimulation and bioengineering are also presented. Additionally, we highlight the challenges and future directions in translating these therapies into clinical practice. Overall, the treatment of ischemic stroke is a complex and multifaceted process that requires a combination of acute measures as well as longer-term strategies to promote brain repair and recovery. The treatment of ischemic stroke has made significant progress in recent years with the development of new treatments and ongoing research to improve outcomes for stroke patients. However, before these therapies can be successfully integrated into routine clinical practise, further research is needed to establish standardised protocols, overcome methodological limitations, and overcome clinical challenges. By further deepening our understanding of the pathophysiology of ischemic stroke and developing innovative treatments, we can improve outcomes and quality of life for stroke survivors.
Keywords
Ischemic stroke, cerebral ischemia, treatment, target, stem cell and gene therapy, neuroregeneration, neuroprotection, rehabilitationIntroduction
Ischemic stroke is a leading cause of mortality and morbidity worldwide, accounting for nearly 80% of all stroke cases [1]. Cerebral ischemia occurs when there is insufficient blood supply to the brain to meet metabolic needs. This leads to a restricted oxygen supply or cerebral hypoxia and results in the death of brain tissue, a cerebral infarction or an ischemic stroke [2, 3]. This can be caused either by a blockage of the blood vessels supplying the brain-ischemic stroke or by a decrease in blood flow due to a decrease in cerebral perfusion pressure, such as in hypotension or shock [2, 3]. Ischemic stroke can be caused by various factors, including arterial occlusion, embolism, or systemic hypoperfusion [2, 3]. Arterial occlusion, which is the most common cause of ischemic stroke, occurs when there is a blockage in one or more of the arteries that supply blood to the brain. This can be due to the formation of a blood clot—thrombosis, or the dislodgement of a clot from another part of the body—embolism [2, 3]. Systemic hypoperfusion, on the other hand, occurs when there is a decrease in blood flow to the brain due to systemic factors such as low blood pressure or heart failure [2, 3]. Once the blood supply to the brain is compromised, a cascade of complex events is initiated, including cell swelling, membrane disruption, release of excitatory neurotransmitters, and activation of inflammatory processes, that ultimately leads to cell death and tissue damage within minutes to hours of the ischemic insult [3]. The pathophysiology of ischemic stroke can be divided into two main phases: the acute phase and the delayed phase (Figure 1). In the acute phase, which occurs within minutes to hours of the onset of ischemic stroke, various mechanisms come into play to try to restore blood flow to the affected brain tissue. These mechanisms include vasodilation, collateral circulation, and neuroprotective responses [4]. However, if the ischemic insult is severe or prolonged, these compensatory mechanisms may fail, leading to cell death and tissue damage.
This review provides a comprehensive overview of the current state of knowledge on acute and longer-term therapeutic targets, and innovative treatments for ischemic stroke.
Treatment modalities in ischemic stroke
Stroke is a leading cause of death and disability worldwide, making early diagnosis and intervention critical in improving patient outcomes [2, 5]. Sex differences in stroke outcomes are notable, with women experiencing higher incidence rates, greater disability-adjusted life years, and poorer recovery compared to men, even after adjusting for age and comorbidities [6].
The management of ischemic stroke typically involves a combination of medical, surgical, and rehabilitative interventions [7]. Over the years, significant advancements have been made in the treatment of ischemic stroke, with the development of new treatment modalities and ongoing research aimed at improving outcomes for ischemic stroke patients.
The primary goal of treatment is to restore blood flow to the affected area of the brain as quickly as possible to minimize and limit neuronal damage and to improve and promote neurological recovery [8]. The following are some of the key treatment modalities used in the management of ischemic stroke.
Recanalization therapies
Recanalization therapies aim to restore blood flow to the ischemic brain tissue by removing or dissolving the obstructing clot.
Endovascular recanalization remains the primary approach encompassing balloon angioplasty, stent implantation, intraarterial thrombolysis, and thrombectomy up to a 24-hour window for stroke patients [9]. Intravenous thrombolysis with tissue plasminogen activator (tPA) is an effective and time-critical treatment for acute ischemic stroke. Mechanical thrombectomy, using endovascular devices, is another recanalization strategy for large vessel occlusions in ischemic stroke. Early intervention with recanalization therapies can salvage brain tissue and improve functional outcomes in ischemic stroke patients [10]. However, recanalization therapies are only applicable to a small proportion of patients.
Intravenous thrombolysis
Intravenous thrombolysis with tPA is the gold standard treatment for acute ischemic stroke. tPA works by dissolving the blood clot that is blocking blood flow to the brain, restoring perfusion, and reducing neurological deficits. It is most effective when administered within 4.5 hours of symptom onset, although recent studies have suggested that it may be beneficial up to 9 hours after onset in select patients [11].
tPA is the only FDA-approved medical treatment for ischemic stroke. However, it comes with some unavoidable limitations, including a short therapeutic window, an extremely short half-life, and poor penetration into large clots. Systemic administration can lead to complications such as haemorrhagic transformation in the brain, and recurrence in the form of a new stroke [11]. Haemorrhagic transformation is the most frequent complication and a significant concern following ischemic stroke, warranting close monitoring and management to optimize patient outcomes. This limitation has motivated researchers to search for additional thrombolytic agents that have a dependence on extensive fibrin selectivity without affecting the brain [12]. Furthermore, ultrasound has been utilised in combination with contrast agents, echogenic liposomes, microspheres, polymeric and magnetic nanoparticles, or an intelligent DNA nanodevice carrying tPA for improving thrombolysis—an approach that has resulted in slight improvement of tPA delivery and facilitated thrombolysis [13].
Most of these delivery systems are able to extend the circulating half-life and clot penetration of tPA [11]. Various technologies employed for ameliorated thrombolytic therapy are in different phases, some are in final steps for clinical applications while others are under investigations for their safety and efficacy in human cases.
In addition, the medications used in thrombolysis can increase the risk of bleeding, particularly in the brain, which can lead to potentially life-threatening complications [14]. For this reason, thrombolysis is only used in carefully selected patients who are at a high risk of permanent damage or death if the clot is not treated [14].
Mechanical thrombectomy
Mechanical thrombectomy is a minimally invasive procedure that involves the removal of the blood clot causing the stroke using a catheter-based device. This treatment is typically performed in conjunction with intravenous thrombolysis and has been shown to significantly improve outcomes in patients with large vessel occlusions. Recent advancements in thrombectomy technology, such as the use of stent retrievers and aspiration devices, have further improved the success rates of this procedure [10].
Anticoagulant therapy
Anticoagulant therapy works by slowing down the clotting process in the blood, reducing the risk of blood clot formation. Anticoagulants work by inhibiting the body’s ability to form clots, thus reducing the risk of blood clots that can lead to serious health complications. Common examples of anticoagulant medications include warfarin, heparin, dabigatran, rivaroxaban, and apixaban [15, 16]. It is important to closely monitor anticoagulant therapy, as too much can lead to excessive bleeding and too little may not provide enough protection against clot formation.
Vasodilators and antithrombotic agents aim to improve blood flow to the affected areas of the brain after an ischemic stroke. These agents help to restore blood flow and reduce the risk of secondary ischemic events. Common vasodilators used in ischemic stroke include nitric oxide donors (e.g., nitroglycerin) and calcium channel blockers (e.g., nimodipine, nicardipine) [17, 18]. Antithrombotic agents such as antiplatelet agents (e.g., aspirin) and anticoagulants (e.g., heparin) can also be used to promote blood flow [19]. Platelet aggregation and the formation of thrombi contribute to the development and worsening of ischemic strokes. Antiplatelet agents such as clopidogrel or ticagrelor inhibit platelet activation and aggregation and thus reduce the risk of further vascular occlusion [19, 20].
Acute stroke units
Acute stroke units are specialized hospital wards dedicated to the care of stroke patients. These units provide multidisciplinary care, including early assessment, detection of complications, and initiation of appropriate treatment. This allows for more personalized and effective treatment for each patient. Studies have shown that patients treated in acute stroke units have better outcomes and lower mortality rates compared to those treated in general medical wards [21].
Rehabilitation
Rehabilitation plays a crucial role in the recovery and functional independence of ischemic and haemorrhagic stroke survivors. Physical therapy, occupational therapy, speech therapy, and cognitive rehabilitation are important components of the multidisciplinary rehabilitation program for stroke patients [22]. Rehabilitation aims to improve motor function, coordination, balance, speech, cognition, and activities of daily living in stroke survivors. Cognitive rehabilitation focuses on improving cognitive function, including memory, attention, and executive function, after a stroke. Cognitive rehabilitation programs include a combination of mental exercises, memory training, problem-solving tasks, and compensatory strategies to help individuals improve their cognitive skills and overcome challenges related to cognitive impairment [10].
Rehabilitation aims to maximise a patient’s intrinsic and adaptive recovery by addressing specific impairments (e.g., weakness), activity limitations (e.g., difficulty walking), reduced social participation (e.g., less contact with friends), and overall quality of life. Rehabilitation should be personalised and take into account not only the problems caused by the stroke but also comorbidities and the patient’s views and preferences [21].
Psychological support is an important part of stroke rehabilitation as people can experience emotional and psychological challenges such as depression, anxiety, and frustration after a stroke. Psychologists, counsellors, and social workers provide counselling, support and education to help people cope with the emotional and psychological impact of stroke and develop coping strategies to improve their mental wellbeing. It is important that individuals work closely with their medical team to develop a personalised rehabilitation plan that addresses their specific needs and goals to achieve the best possible outcomes on their road to recovery. Early initiation of rehabilitation and intensive therapy has been shown to improve recovery and functional independence in patients with ischemic stroke [22].
Current advancements in treatments of ischemic stroke
In recent years, there have been several significant advancements in the treatment of ischemic stroke aimed at improving outcomes and reducing disability [8]. Some of the key advancements include.
Extended time windows for thrombolysis
Advanced technologies are implemented for efficient delivery of tPA and advanced thrombolytic therapy [13, 23]. Recent clinical trials, such as the DAWN and DEFUSE-3 trials, have demonstrated that select patients with large vessel occlusions can benefit from thrombolytic therapy up to 24 hours after symptom onset [24].
These findings have led to a paradigm shift in the management of acute ischemic stroke, with more patients now eligible for thrombolysis beyond the traditional time window of 4.5 hours.
Mobile stroke units
Mobile stroke units (MSU) are specialised ambulances equipped with staff, advanced imaging technology, and telemedicine capabilities to diagnose and treat stroke patients before they are admitted to hospital. These units have been shown to significantly reduce treatment times and improve outcomes for patients with acute ischemic stroke [25]. Clinical trials have shown that treatment with MSUs was safe compared to conventional emergency care and resulted in better functional outcomes for stroke patients [25]. Questions remain about the cost-effectiveness of MSUs, and their utility in rural areas [25].
Telerehabilitation
Telerehabilitation is a novel approach to delivering rehabilitation services to stroke survivors remotely using telecommunication technology. This allows patients to receive therapy in the comfort of their own homes, reducing the burden of travel and increasing access to care. Studies have shown that telerehabilitation is effective in improving functional outcomes and quality of life in stroke survivors [26].
Neuroimaging biomarkers
Mimics account for almost half of hospital admissions for suspected stroke [27]. Stroke mimics may present as a functional (conversion) disorder or may be part of the symptomatology of a neurological or medical disorder [27]. Accurate diagnosis is important as recurrent presentations may be common in many disorders [27].
Neuroimaging biomarkers play a crucial role in the assessment and management of stroke patients, providing valuable insights into the underlying pathophysiology. Advances in neuroimaging technology have led to the identification of novel biomarkers that can predict the severity of ischemic stroke, guide treatment decisions, and monitor disease progression.
One of the most commonly used imaging techniques for the assessment of strokes is computed tomography (CT). CT scans can quickly detect the presence of a stroke, differentiate between ischemic and haemorrhagic strokes and assess the extent of damage to brain tissue [27]. However, a non-contrast CT is not sufficient to make a diagnosis of acute stroke as the test may be normal very early following an acute stroke. Multi-modal CT or magnetic resonance imaging (MRI) may be helpful to confirm an acute ischemic stroke and is necessary if stroke mimics are suspected [27]. CT perfusion imaging provides information about cerebral blood flow and helps predict tissue viability [27].
MRI is another valuable tool in the assessment of stroke patients. It offers better soft tissue contrast and the ability to visualise ischemic changes in the brain with greater sensitivity than CT [27, 28]. Diffusion-weighted imaging (DWI) is particularly useful for the early detection of ischemic stroke, as it reveals restricted diffusion in affected brain tissue within minutes of the onset of symptoms [29]. Perfusion-weighted imaging (PWI) can assess the extent of tissue at risk [29].
Advanced MRI techniques such as functional MRI (fMRI) and diffusion tensor imaging (DTI) can provide additional insights into the functional and structural connectivity of the brain after stroke [30]. fMRI can map brain activity and identify areas of neuronal plasticity and recovery, while DTI can assess white matter integrity and track changes in connectivity after stroke [31]. These techniques help predict functional outcomes and determine rehabilitation strategies for stroke patients.
Other imaging biomarkers that have shown promise for stroke assessment are positron emission tomography (PET) and single photon emission CT (SPECT) [30]. These modalities provide information on cerebral metabolism, blood flow, and neurotransmitter function, shedding light on the underlying pathophysiology of stroke and enabling targeted therapies [32, 33].
Machine learning and artificial intelligence algorithms are increasingly being applied to neuroimaging data in stroke research, offering the potential to identify novel biomarkers and improve diagnostic accuracy. These techniques analyse large datasets of imaging features and clinical data to predict outcomes, stratify patient risk, and personalize treatment approaches in stroke patients [31].
Cellular and molecular therapeutic targets in ischemic stroke
Understanding the complex pathophysiology of ischemic stroke is crucial for developing effective treatment strategies to prevent or minimize brain damage. Various treatment modalities have been developed to target these underlying cellular and molecular mechanisms in ischemic stroke.
Energy failure
A critical consequence of impaired blood flow is the loss of energy production, known as cerebral metabolic distress, in the affected brain regions. This leads to a lack of energy production through glycolysis, the first step of glucose metabolism [34, 35]. In addition, the lack of oxygen that reaches brain cells during an ischemic stroke prevents efficient energy production through oxidative phosphorylation, the process by which cells generate energy in the form of adenosine triphosphate (ATP) in the mitochondria [34]. Without an adequate supply of oxygen, brain cells are unable to produce energy efficiently, leading to a state of energy failure [35].
The consequences of energy failure in ischemic stroke are significant. ATP is essential for maintaining normal cellular functions such as ion homeostasis, the release of neurotransmitters, and the maintenance of cell membrane potential [34]. When ATP levels drop due to energy deficiency, these essential cellular processes are impaired, leading to cellular dysfunction and ultimately cell death [35]. There are several strategies to mitigate energy failure in ischemic stroke. One approach is to restore blood flow to the affected brain regions through reperfusion therapies such as intravenous thrombolysis or mechanical thrombectomy [8]. Another strategy is to improve cellular energy production by administering metabolic substrates such as glucose or ketone bodies (e.g., β-hydroxybutyrate-BHB). These substances can bypass the impaired glycolytic and oxidative phosphorylation pathways and provide brain cells with an alternative energy source [36].
Cellular acidosis
In addition, an ischemic stroke also leads to cellular acidosis, i.e. an imbalance in the pH levels of the cells. When the blood supply to the brain is blocked, the cells are unable to produce energy through aerobic metabolism and must rely on anaerobic metabolism, which produces lactic acid as a by-product. This lactic acid can accumulate in the cells, lowering their pH and causing cellular acidosis. Acidosis can have several detrimental effects on cells, including disrupting ion channels, impairing protein function, and promoting cell death [37].
A possible therapeutic approach is to neutralise cellular acidosis. Studies have shown that acidosis exacerbates brain damage in ischemic stroke, while alkalisation of the brain can have a neuroprotective effect [37]. Alkalising agents such as bicarbonate can help restore normal pH in cells and reduce the harmful effects of acidosis [37]. In addition, targeting specific ion channels involved in acidosis-induced cell death (e.g., ASIC1a, TRPV1, ASIC3, ASIC2, ASIC5) may have a neuroprotective effect in ischemic stroke [37].
Excitotoxicity
Excitotoxicity is a key pathological mechanism and plays a significant role in the pathogenesis of ischemic stroke [38]. Excitotoxicity refers to the overactivation of excitatory neurotransmitter receptors, particularly the N-methyl-D-aspartate (NMDA) receptor, in response to ischemic insult. NMDA receptors are important as they are calcium-permeable channels that can lead to an influx of calcium ions into neurons, ultimately leading to cell death. Excessive activation of these receptors leads to an influx of calcium ions into the neurons, causing cell swelling, mitochondrial dysfunction, and eventually cell death and dysfunction [39]. Targeting excitotoxicity pathways has the potential to reduce brain damage and improve outcomes in ischemic stroke.
Glutamate receptors overactivation
Neuroprotective drugs have been developed that block the presynaptic and postsynaptic glutamate receptors. Targeting glutamate receptors with antagonists or modulators can help prevent neuronal damage and improve outcomes. Inhibiting the activity of NMDA receptors, AMPA or kainate receptors has been shown to reduce brain damage and improve functional recovery in animal models of cerebral ischemia [17, 40, 41]. However, these treatments have not shown efficacy in human clinical trials, so the search for new therapeutic strategies is urgently needed [41].
Calcium channels dysregulation
Dysregulation of intracellular calcium levels is another key mechanism underlying neuronal cell death in ischemic stroke. Calcium ions play a crucial role in neuronal function as they act as second messengers to regulate various cellular processes. For example, calcium ions can stimulate the release of neurotransmitters from synaptic vesicles, which is essential for neuronal communication. They can also activate various kinases and phosphatases that are involved in gene expression, synaptic plasticity, and cell survival [42].
The mitochondria and endoplasmic reticulum (ER) are organelles that regulate intracellular calcium homeostasis via various calcium channels, e.g., the mitochondrial calcium uniporter (MCU), the mitochondrial Na+/Ca2+ exchanger (NCLX), voltage-dependent anion channels (VDACs), ryanodine receptors (RyRs), sarco/ER calcium ATPases (SERCAs), and inositol trisphosphate receptors (IP3Rs) [42].
Mitochondrial dysfunction
The mitochondria, the power plants of the cell responsible for energy production, play a crucial role in the pathophysiology of ischemic stroke. Mitochondria play a central role in cell survival and death pathways and regulate processes such as apoptosis, pyroptosis, necroptosis, ferroptosis, necrosis, and autophagy [9, 43, 44]. There is increasing evidence that mitophagy and ferroptosis play a crucial regulatory role in stroke progression [45, 46].
The release of cytochrome c from damaged mitochondria can trigger apoptotic processes that lead to cell death [47]. In addition, mitochondrial dynamics, including fission and fusion, can be altered in response to ischemic stroke, further impairing mitochondrial function and cell survival [47, 48]. Another important aspect is mitophagy mediated by mitochondria to remove inactivated or damaged mitochondria [43]. While mitochondria scavenge damaged mitochondria, effective promotion of mitophagy can remove damaged mitochondria in a timely manner, preventing apoptosis and reducing ischemia-reperfusion injury [9].
Most cellular stress responses are centred on the mitochondria. Consequently, mitochondria must respond rapidly to maintain cellular homeostasis and physiological requirements by fine-tuning a variety of mitochondria-related processes. The outer mitochondrial membrane (OMM) proteins play a central role in mediating mitochondrial dynamics associated with continuous fission and fusion. These OMM proteins also play an important role in the control of mitochondrial quality and serve as mitophagic receptors for autophagosome entrapment during mitophagy [49].
Cellular responses to stroke include autophagy as an adaptive mechanism that alleviates cellular stress by removing long-lived or damaged organelles, protein aggregates, and excess cellular components via the autophagosome-lysosome degradation process. In this context, autophagy functions as an essential cellular process to maintain cellular homeostasis and survival [50, 51].
Therapeutic strategies targeting mitochondrial function in ischemic stroke are currently being explored. These include the use of mitochondrial protective agents such as antioxidants and modulators of mitochondrial membrane potential as well as strategies to improve mitochondrial biogenesis and autophagy [51].
Endoplasmic reticulum stress
The ER is a network of membranes in cells that is responsible for the synthesis, folding, and transport of proteins [52]. During an ischemic stroke, the ER is stressed and can no longer function properly. This lead to the accumulation of misfolded proteins that trigger a signalling pathway known as the unfolded protein response (UPR). The UPR is a cellular stress response mechanism that aims to restore ER homeostasis by reducing protein synthesis, increasing protein folding capacity, and degrading misfolded proteins. However, if the stress is too severe or too prolonged, the UPR can switch from a pro-survival response to a pro-apoptosis response, leading to cell death [52].
One potential therapeutic approach is to modulate the UPR to enhance the pro-survival response while suppressing the pro-apoptotic response. For example, small molecules known as chemical chaperones can help fold misfolded proteins and alleviate ER stress [53].
Additionally, drugs that target specific UPR signalling pathways to promote cell survival are:
PERK inhibitors: Protein kinase R (PKR)-like ER kinase (PERK) is a key regulator of the UPR pathway. Inhibitors targeting PERK can promote cell survival by preventing excessive UPR activation and subsequent cell death [52, 54].
IRE1 inhibitors: Inositol-requiring enzyme 1 (IRE1) is another essential component of the UPR pathway. Inhibitors targeting IRE1 can promote cell survival by preventing the splicing of X-box binding protein 1 (XBP1) mRNA and subsequent activation of pro-apoptotic pathways [55].
ATF6 activators: Activating transcription factor 6 (ATF6) is a transcription factor that is activated in response to ER stress. Activators of ATF6 can promote cell survival by enhancing the adaptive UPR response and promoting the expression of pro-survival genes [56].
CHOP inhibitors: C/EBP homologous protein (CHOP) is a pro-apoptotic transcription factor that is induced during ER stress. Inhibitors targeting CHOP can promote cell survival by preventing the upregulation of pro-apoptotic genes and pathways [54].
XBP1 activators: XBP1 is a key transcription factor involved in the UPR pathway. Activators of XBP1 can promote cell survival by enhancing the adaptive UPR response and promoting the expression of genes involved in ER stress recovery [57].
Studies have shown that ER stress markers are elevated in the brains of stroke patients and animal models of stroke [36, 52].
Oxidative stress
Oxidative stress is another key pathological mechanism in ischemic stroke, characterized by the reactive oxygen species (ROS), nitric oxide, and free radicals production and the impairment of antioxidant defence systems. Under ischemic conditions, oxidative stress contributes to lipid peroxidation, protein oxidation, DNA damage, mitochondrial dysfunction, inflammation, and cell death [58, 59].
Increased production of nitric oxide—nitrosative stress, triggers further brain damage after ischemic stroke through enhancing oxidative stress and free radical formation. Excessive reactive nitrogen species (RNS) and ROS production cause oxidative stress which damages tissues and cells while interfering with signalling pathways [12]. This damage can significantly compromise cell viability and health and prompt several cellular responses by generating secondary reactive species, eventually triggering cell death via apoptosis or necrosis [12].
Additionally, targeting specific antioxidant enzymes such as superoxide dismutase (SOD) or catalase has been shown to reduce oxidative damage and improve outcomes in cerebral ischemia models [60].
N-acetylcysteine (NAC) is another antioxidant that can help protect the brain from oxidative stress during ischemic stroke. It can also help reduce inflammation and promote cell survival as it was proven in a randomized controlled pilot trial NACTLYS [61]. Edaravone is a free radical scavenger that helps reduce oxidative stress and inflammation in the brain. Studies have shown that edaravone can improve neurological outcomes and reduce brain damage in patients with acute ischemic stroke. It is usually administered intravenously and is well-tolerated with minimal side effects [62]. NXY-059 is another neuroprotective agent that has been studied in ischemic stroke. NXY-059 is a free radical scavenger that has shown promise in reducing oxidative stress and inflammation in the brain [63]. However, clinical trials have produced mixed results, with some studies showing a benefit in improving outcomes in acute stroke patients, while others have not shown a significant effect.
One of the therapeutic targets in oxidative stress is the nuclear factor E2-related factor 2, Nrf2-ARE pathway, which regulates the expression of antioxidant genes in response to oxidative stress. Activation of Nrf2 has been shown to protect against ischemic brain injury and improve functional recovery in animal models of cerebral ischemia [64].
Blood-brain barrier disruption
One of the key mechanisms in the pathophysiology of ischemic stroke is the disruption of the blood-brain barrier (BBB). The BBB is a highly selective cellular monolayer found only in the microvasculature of the central nervous system (CNS). It mediates the communication of the CNS with the rest of the body by regulating the passage of molecules into the CNS microenvironment. The restriction of the passage of substances through the BBB is mainly due to the tight junctions and adherens junctions between the microvascular endothelial cells of the brain [65, 66].
The BBB consists of brain endothelial cells connected by tight junctions, astrocytes, pericytes, neurons, supporting structures (microglia, oligodendrocytes), and the extracellular matrix. It acts as a selective barrier that regulates the exchange of substances between the bloodstream and the brain parenchyma and prevents the passage of most molecules, ions, and cells from the blood into the brain, while the neurovascular unit (NVU) comprises the BBB together with the neurons and the surrounding extracellular matrix [9, 67–69].
The disruption of the BBB may also be caused by the activation of matrix metalloproteinases (MMPs) in response to the ischemic insult. MMPs are enzymes that degrade the extracellular matrix surrounding blood vessels and endothelial cells, leading to increased BBB permeability [70]. It has been confirmed that MMP-2, MMP-3, and MMP-9 are involved in the destruction of the BBB and NVU [9, 70, 71].
The breakdown of the BBB during a stroke has several detrimental effects on the brain. Firstly, it causes blood components such as plasma proteins and red blood cells to extravasate into the brain parenchyma. These components cause inflammation, oxidative stress, and further damage to brain tissue [69, 72].
The increased permeability of the BBB also allows immune cells to enter the brain, further exacerbating the inflammatory response and tissue damage. This leads to the recruitment of more inflammatory cells, the release of more pro-inflammatory cytokines, and ultimately to a vicious cycle of inflammation and tissue damage [68].
In addition, the impaired BBB hinders the supply of important nutrients and therapeutic agents to the brain. For example, BBB permeability can prevent neuroprotective drugs or stem cells from reaching the site of injury, limiting their therapeutic potential in the treatment of stroke [73, 74].
Neuroinflammation
Neuroinflammation plays a crucial role in the pathophysiology of ischemic stroke and contributes to secondary brain damage and functional impairment [75] (Figure 1). Ischemic stroke triggers an inflammatory response in the brain in which microglia and astrocytes are activated and immune cells from the periphery invade the brain tissue. The release of pro-inflammatory cytokines, chemokines, and adhesion molecules contributes to the recruitment of immune cells and exacerbates the neuroinflammatory response [75, 76].
The acute neuroinflammation occurs immediately after an ischemic stroke and lasts for several days. Cytokines and chemokines promote the migration of neutrophils and macrophages to the centre of inflammation in the brain [77]. Neuroinflammation that lasts 2–6 weeks is referred to as subacute neuroinflammation, while chronic post-ischemic inflammation lasts months or years [77, 78]. In chronic neuroinflammation, macrophages, lymphocytes, and plasma cells predominate, in contrast to neutrophils, which predominate in acute neuroinflammation [77]. In addition to their detrimental effect on the ischemic brain, inflammatory mediators may also have positive effects on recovery from stroke [79]. The role of secondary inflammatory cells in the pathophysiology of ischemic stroke is still less understood. Late post-ischemic neuroinflammation leads to secondary damage of neuronal cells. The inflammatory response can further exacerbate neuronal death and tissue damage, leading to a self-repetitive cycle of damage [77].
Key strategies for the treatment of ischemic stroke include targeting inflammatory responses, immunomodulation (particularly of immune cells), and inflammatory responses to suppress stroke progression [80]. For example, one potential therapeutic target is nuclear factor kappa B (NF-κB), which regulates the expression of pro-inflammatory genes in response to ischemic injury. Inhibition of NF-κB activation has been shown to reduce brain injury and improve functional recovery in animal models of cerebral ischemia [80]. Similarly, targeting specific cytokines such as tumor necrosis factor-alpha (TNF-α) or interleukin-1 beta (IL-1β) has been shown to attenuate neuroinflammation and protect against ischemic brain damage [80].
Apoptosis
In the delayed phase of ischemic stroke, which occurs hours to days after the initial insult, secondary injury mechanisms come into play that further exacerbate the damage to the brain tissue (Figure 1). In response to an ischemic insult, apoptotic signalling pathways are activated that lead to DNA fragmentation and cell death. One of the most important pathways is the mitochondrial pathway, which is initiated by the release of cytochrome c from the mitochondria into the cytoplasm. Cytochrome c then activates the caspases, a family of proteases that play a crucial role in the execution of apoptosis. The activation of caspases leads to the cleavage of various cellular proteins, which ultimately leads to cell death [81].
The consequences of apoptosis in stroke are profound. The death of cells in the brain leads to a loss of function in the affected area, resulting in motor, sensory, and cognitive deficits [3]. In addition, the release of cell contents during apoptosis can trigger an inflammatory response that further exacerbates tissue damage and contributes to secondary injury [77].
Targeting apoptotic signalling pathways with inhibitors or modulators helps to prevent neuronal cell death and promote neuroprotection. Several potential approaches have been investigated, including the use of caspase inhibitors to block the activation of caspases and prevent cell death [81]. Other approaches target specific signalling pathways involved in apoptosis, such as the PI3K/Akt pathway, which has been shown to have neuroprotective effects [82]. Another therapeutic target is the Bcl-2 family of proteins, which regulates the mitochondrial pathway of apoptosis in response to ischemic injury. Modulation of members of the Bcl-2 family has been shown to reduce neuronal cell death and improve functional recovery in animal models of stroke [83]. In addition, influencing other apoptotic signalling pathways such as the extrinsic signalling pathway or the ER stress pathway has been shown to attenuate apoptosis and protect against ischemic brain injury [55].
Netosis
Netosis is a form of cell death in which chromatin and antimicrobial proteins are expelled from neutrophils, forming so-called neutrophil extracellular traps (NETs) [84]. These NETs play a role in the immune response by trapping and killing pathogens, but they can also contribute to inflammation and tissue damage [84]. The formation of NETs can exacerbate inflammation in the affected brain region and potentially lead to further neuronal damage [84]. The release of NETs can contribute to disruption of the BBB, which can lead to increased permeability and edema [84]. This may worsen the outcome of the stroke by facilitating additional brain injury. NETs can promote immunothrombosis, which is critical in the context of stroke [85]. They can form a scaffold for the accumulation of platelets and fibrin, leading to pathological thrombus formation including venous thrombosis [86]. However, it still remains unclear whether NETs are present in the brain tissue of stroke patients, since all results are obtained in animal models of stroke. Research into the exact mechanisms and effects of netosis in ischemic stroke is ongoing, but evidence to date suggests that it plays a complex and potentially deleterious role in the pathology of this neurovascular event. Understanding the role of netosis in ischemic stroke may open new avenues for therapeutic interventions aimed at modulating the immune response or inhibiting the formation of NETs and thus reducing the inflammatory damage associated with stroke.
Ischemia-reperfusion injury
Another important mechanism in the delayed phase of ischemic stroke is reperfusion injury of the blood. Reperfusion injury occurs after an ischemic stroke when blood flow to the brain is temporarily interrupted and then restored. When blood flow is restored, the sudden influx of oxygen leads to further damage through increased production of ROS and inflammation. This leads to further damage to brain tissue and exacerbates neurological deficits, cognitive impairment, and neurodegeneration in patients with ischemic stroke [87]. The severity of brain damage due to ischemia-reperfusion depends on the duration of blood flow interruption, the extent of tissue damage, and the effectiveness of reperfusion treatment [87].
The pathophysiology of ischemia-reperfusion brain injury is complex and involves multiple mechanisms such as excitotoxicity, oxidative stress, inflammation, and mitochondrial dysfunction [87]. In addition, reperfusion injury leads to calcium overload in the cells, which can disrupt cellular homeostasis and activate cell death pathways. This process, known as calcium-induced cell death, can further contribute to tissue damage and dysfunction [87].
Reperfusion injury is a major challenge in the treatment of ischemic stroke and a target for therapeutic interventions aimed at minimising brain damage. Treatment options for ischemia-reperfusion injury are limited, but efforts are focused on strategies to reduce the damage caused by reperfusion. Treatment strategies for ischemia-reperfusion brain injury focus on minimising tissue damage during the ischemia phase and promoting tissue repair during the reperfusion phase. Therapies such as thrombolytics, neuroprotective drugs, and hypothermia are used to reduce the extent of tissue damage during the ischemic phase [88, 89]. Strategies to promote tissue repair during the reperfusion phase include antioxidants, anti-inflammatory agents, and cell-based therapies to enhance tissue regeneration and repair [90].
Researchers are also exploring experimental therapeutic approaches such as ischemic preconditioning and postconditioning, in which brain tissue is briefly exposed to ischemia before or after the actual ischemic insult. These techniques have been shown to have a protective effect against ischemia-reperfusion injury by activating cellular stress responses and promoting tissue survival [91–94].
Acute and longer-term therapeutic targets for neuroprotection in ischemic stroke
Neuroprotection in ischemic stroke refers to strategies aimed at preserving brain function, preventing further damage, and promoting recovery after a stroke [95–97].
One acute approach to neuroprotection in ischemic stroke is hypothermia. Hypothermia involves lowering body temperature to reduce the metabolic demand of brain cells and protect them from damage during a stroke. This can help to reduce the extent of brain damage and improve outcomes after a stroke [85, 86]. In some cases, initiating therapeutic hypothermia in stroke patients can help reduce brain swelling, protect against further damage, and improve overall outcomes. However, it is important to carefully monitor and control the patient’s body temperature during this process to avoid complications such as shivering, blood pressure changes, or electrolyte imbalances [88, 89].
Longer-term therapeutic targets for neuroprotection in ischemic stroke focus on strategies to promote brain repair and recovery in the days, weeks, and months following a stroke. Neuroplasticity, the brain’s ability to reorganise itself and form new neuronal connections, plays a crucial role in post-stroke recovery [95, 98].
The use of acute and longer-term therapeutic targets for neuroprotection offers a comprehensive approach to improving outcomes for stroke patients. Recanalization therapy, neuroprotective drugs, rehabilitation therapy, and new therapies such as stem cell transplantation all play an important role in preserving brain function and promoting recovery after stroke. Given the time-critical nature of strokes, the dynamics of patients’ conditions fluctuate depending on the timing [99]. The combination of several neuroprotective drugs or treatments often leads to synergistic effects compared to single therapies, e.g., thrombolysis + neuroprotectors, endarterectomy + neuroprotectors, therapeutic combinations between growth and/or trophic factors, etc. [9].
Despite numerous studies, preclinical and clinical research has not yet produced an agent that mitigates the consequences of stroke beyond thrombolysis and mechanical removal of blood clots. These implementation gaps have not deterred the scientific community, as new agents are constantly being investigated. The NIH has recently promoted the concept of cerebroprotection to address the whole brain after stroke, not just the neurons [99]. Neuroprotective treatment research needs to move from protecting neurons to protecting the brain. Neuroprotective treatment should adopt multi-target and multi-pathway treatment and involve all cells of the NVU including neurons involved in the ischemic cascade [9].
So far, only a few neuroprotective agents have been used clinically, as the results of animal studies and clinical trials differ greatly [9]. New animal models adapted to humans are needed for preclinical studies. Most existing animal models, especially rodents, differ from humans in terms of age, thrombosis mode, vascular anatomy, living environment, etc. [9]. It is clear that even primates, beings closely similar to humans, do not fully replicate the environment of the human brain. This could be due to the differences in anatomical structures and the incongruence between surgically induced strokes and common causes of stroke [9].
In addition, Wang et al. [100] successfully produced three-dimensional cerebral organoids from human pluripotent stem cells, which showed similar effects to the traditional models. They tested this new model with recognised neuroprotective drugs such as butylphthalide and edaravone. However, the lack of NVU, BBB, and immune cells in cerebral organoids poses a significant obstacle to the application of this model [9].
Further research and clinical trials are needed to advance neuroprotection strategies and ultimately improve the quality of life of stroke survivors.
Neuroregenerative approaches in ischemic stroke
Stem cell therapy and gene therapy are promising approaches to improve outcomes in ischemic stroke by promoting neuroprotection, neuroregeneration, and angiogenesis.
Stem cell therapy for ischemic stroke
Stem cells are undifferentiated cells with the ability to self-renew and differentiate into different cell types, making them ideal candidates for tissue repair and regeneration. After transplantation, stem cells can migrate and colonise injured areas of the brain, a process that is due to the expression of growth factors and chemokines [101]. Once at the site of injury, the stem cells differentiate into host tissue cells and replace the injured and necrotic neuronal tissue [101]. Through their paracrine mechanisms, stem cells further reduce the injury and stimulate endogenous cells to repair and restore the normal function of neurons [101, 102].
Several types of stem cells have been investigated for their potential therapeutic effect in ischemic stroke, including embryonic stem cells (ESCs), mesenchymal stem cells (MSCs), induced pluripotent stem cells (iPSCs), and neural stem cells (NSCs) [103, 104]. Stem cell therapy involves the transplantation of stem cells into the brain to promote tissue repair and regeneration. Stem cells have the ability to differentiate into neurons, glial cells, and vascular cells, making them a promising candidate for the treatment of ischemic stroke [104].
Due to their immunomodulatory, anti-inflammatory, and trophic effects as well as their promotion of neurogenesis and angiogenesis, MSCs are the most studied stem cell type for ischemic stroke therapy [105]. MSCs can be easily isolated from various sources, including bone marrow, adipose tissue, and umbilical cord blood, making them accessible for clinical use [106, 107]. Preclinical studies have shown that MSCs can improve neurological function, reduce infarct size and promote neurovascular remodelling in animal models of ischemic stroke [108].
Clinical trials investigating the safety and efficacy of MSC therapy for ischemic stroke have shown promising results. A phase I/II clinical trial showed that intravenous infusion of allogeneic MSCs was safe and well tolerated in patients with ischemic stroke, with no serious adverse events reported [103, 109]. The study also showed improvements in neurological function and quality of life in patients receiving MSC therapy compared to controls [103, 109]. These results speak to the potential of MSC therapy as a novel treatment approach for ischemic stroke. However, it remains a challenge to optimise the administration, dosing, and timing of MSC therapy to maximise its therapeutic potential. In addition, the mechanisms underlying the beneficial effects of MSC therapy in ischemic stroke are not yet fully understood and require further investigation. MSCs have shown a low survival rate, limited grafting into damaged areas, and low differentiation into fully functional tissue [102, 110]. Studies suggest that their potential beneficial effect is primarily due to their trophic effect rather than the replacement of damaged or lost brain tissue [102, 109, 110].
NSCs are another promising stem cell type for the treatment of ischemic stroke, as they can differentiate into neurons and glial cells, restore damaged brain tissue, and promote neurogenesis [102, 103]. They originate mainly from the subventricular zone and the subgranular zone of the dentate gyrus of the hippocampus. During embryonic development of the CNS, NSCs give rise to neurons, astrocytes, and oligodendrocytes [102, 103].
Preclinical studies have shown the therapeutic potential of NSCs in ischemic strokes, as they can integrate into the host brain, differentiate into functional neurons, and improve synaptic plasticity [102, 103]. However, NSC therapy faces challenges such as limited availability, ethical concerns regarding its use, and the potential for tumourigenicity [102, 103].
iPSCs have great potential for the treatment of ischemic stroke and could open a new avenue for regenerative medicine approaches to improve patient outcomes and quality of life. By differentiating iPSCs into nerve cells, researchers can generate new neurons and other supporting cells to replace those lost due to stroke [104]. In addition, iPSCs have the advantage that they can be personalised for each individual patient, reducing the risk of immune rejection [103, 104]. Studies have shown promising results in preclinical models of ischemic stroke, with iPSCs being able to improve functional outcomes and reduce the size of infarcted areas in the brain [103, 104]. However, further research is needed to address safety concerns, optimise the delivery of iPSCs to the brain and improve the efficiency of differentiation into neurons.
Gene therapy for ischemic stroke
Gene therapy involves the delivery of genes or genetic material into target cells or tissues to modulate gene expression, protein production, and cellular functions to promote tissue repair and regeneration [111]. Gene therapy for ischemic stroke aims to improve neuroprotection, promote tissue repair neuroregeneration, and angiogenesis, and modulate inflammatory responses by manipulating specific genes and signalling pathways by targeting specific molecular pathways [111]. Several gene therapy approaches have been investigated for their potential therapeutic effect in ischemic stroke, including gene therapy with neurotrophic factors, anti-inflammatory cytokines, growth factors, and stem cell-specific genes [111].
Pharmacological interventions targeting neurogenesis, angiogenesis, and synaptogenesis may also promote brain repair and recovery in patients with ischemic stroke. Neurotrophic factors such as brain-derived neurotrophic factor (BDNF), glial cell line-derived neurotrophic factor (GDNF), and nerve growth factor (NGF) have shown neuroprotective and neurotrophic effects in animal models of ischemic stroke, promoting neuronal survival, synaptic plasticity, repair, and functional recovery [112, 113]. Targeting these factors through exogenous administration or gene therapy may support neuronal recovery after ischemic stroke.
One of the most promising gene therapy approaches for ischemic stroke is the delivery of the vascular endothelial growth factor (VEGF) gene. VEGF is a potent angiogenic factor that promotes the growth of new blood vessels and improves blood flow to ischemic brain tissue [114, 115]. Preclinical studies have shown that VEGF gene therapy can improve neurological function, reduce infarct size and promote neurovascular remodelling in animal models of ischemic stroke [114, 115]. VEGF gene therapy can be delivered using viral vectors, non-viral vectors, or cell-based approaches, with the potential for sustained expression of the therapeutic gene in the brain [116, 117].
Clinical trials investigating the safety and efficacy of gene therapy for ischemic stroke are currently underway. A phase I/II clinical trial demonstrated the safety and feasibility of VEGF gene therapy in patients with chronic cerebral ischemia. The study showed improvements in neurological function, cerebral blood flow, and metabolic activity in patients receiving VEGF gene therapy compared to controls [118]. These results support the potential of gene therapy as a novel treatment approach for ischemic strokes.
The combination of stem cell therapy and gene therapy in the treatment of ischemic stroke offers synergistic effects by targeting multiple signalling pathways involved in tissue repair and regeneration. Stem cells can be a source of trophic factors and cellular support for gene therapy approaches, while gene therapy can improve the survival, differentiation, and function of transplanted stem cells in ischemic brain tissue. By transducing stem cells with specific genes such as growth factors or neurotrophic factors, researchers can improve their paracrine signalling, immunomodulatory properties, and their ability to promote tissue repair and regeneration [104]. Preclinical studies have shown that the combination of stem cell therapy and gene therapy can improve outcomes in animal models of ischemic stroke, leading to enhanced neuroprotection, neuroregeneration, and angiogenesis.
Gene editing technologies such as CRISPR-Cas9 have revolutionised the field of gene therapy by enabling precise modification of the genome, correction of genetic mutations, and targeted gene expression [119]. These technologies hold great promise for the development of novel gene therapy approaches for ischemic stroke, including correction of genetic risk factors, modulation of disease-related signalling pathways, and enhancement of endogenous repair mechanisms.
Clinical trials investigating the safety and efficacy of these novel therapies have shown promising results, supporting their potential as new treatment options for ischemic stroke.
Challenges and future directions for stem cell and gene therapy in ischemic stroke
Despite the promising results of stem cell and gene therapy for ischemic stroke, there are still some challenges in translating these treatments from preclinical studies into clinical applications. These challenges include ethical issues, the type of stem cells used, the optimal method and route of administration, dosing—the number of stem cells administered and timing of therapy—the preconditioning and the injection schedule, clarifying safety concerns such as tumourigenicity and immune responses, and understanding the mechanisms underlying the therapeutic effects of stem cells and gene therapy to maximise therapeutic benefits and minimise risks [102]. The long-term safety of stem cell treatment and the age of the recipient also need to be further investigated [102].
Future research directions in stem cell and gene therapy for ischemic stroke include the development of novel stem cell sources, optimisation of gene delivery systems, identification of biomarkers for patient selection and monitoring, and integration of stem cell and gene therapy with other treatment modalities such as rehabilitation and neuroprotective agents.
The lack of translational implementation could be explained by several aspects: quality of studies, type of animal model, therapeutic modalities, timing, preconditioning, etc. [102, 120]. High-quality preclinical studies should address factors such as the optimal cell type, the amount of stem cells administered, the route of administration, underlying comorbidities, and the timing of administration. In addition, it is important to ensure that preclinical studies are comprehensive and well designed to provide solid evidence of the safety and efficacy of stem cell therapy [102].
Advances in stem cell technology, gene delivery systems, and imaging techniques will further improve the development and translation of these therapies into clinical practise. Personalised medicine approaches, such as stratification of patients based on genetic profiles and imaging biomarkers, will also help to adapt treatment strategies for individual patients with ischemic stroke. Collaboration between researchers, clinicians, and industry partners is essential to advance the field of regenerative medicine and develop effective therapies for ischemic stroke.
New treatment directions for ischemic stroke
Despite recent advances in the treatment of ischemic stroke, there are still many challenges and unanswered questions in this field. Ongoing research is focussed on addressing these issues and developing new therapies to improve outcomes for stroke patients. Some of the key areas of ongoing research in the field of ischemic stroke are (Figure 2).
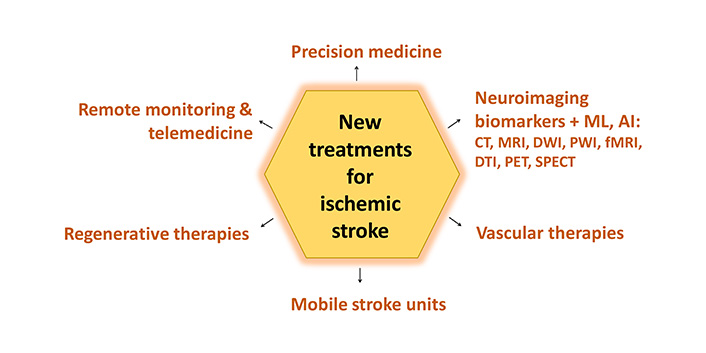
New treatments for ischemic stroke. ML: machine learning; AI: artificial intelligence; CT: computed tomography; MRI: magnetic resonance imaging; DWI: diffusion-weighted imaging; PWI: perfusion-weighted imaging; fMRI: functional MRI; DTI: diffusion tensor imaging; PET: positron emission tomography; SPECT: single photon emission CT
Precision medicine
Precision medicine aims to tailor treatment strategies to individual patient characteristics such as age, gender, lifestyle, genetic predisposition, comorbidities, and response to therapy. By identifying biomarkers that predict treatment response and prognosis, clinicians can optimise treatment strategies and improve outcomes for stroke patients. Ongoing research is focused on identifying new biomarkers and developing personalised treatment algorithms for ischemic stroke [121].
Vascular therapies
Vascular therapies aim to optimise blood flow to the brain and promote neurovascular repair after an ischemic stroke. Approaches such as promoting angiogenesis, vasodilation, and BBB protection have shown promise in preclinical studies and are currently being translated into clinical trials [122]. These therapies have the potential to improve tissue perfusion, stimulate neurogenesis, and improve functional outcomes in stroke patients.
Remote monitoring and telemedicine
Remote monitoring and telemedicine technologies enable continuous monitoring of stroke patients’ vital signs, neurological condition, and response to out-of-hospital treatment. This enables early detection of complications, timely intervention, and personalised care, which ultimately improves treatment outcomes and reduces healthcare costs. Ongoing research, a telemedicine-oriented software system called TeleStroke, and pilot trials are focused on integrating remote monitoring and telemedicine into routine stroke care to improve patient outcomes [123, 124].
Regenerative therapies
Regenerative therapies aim to repair and regenerate damaged brain tissue, promote neuroplasticity, and restore lost neurological function after a stroke. These therapies utilise the body’s natural healing mechanisms to promote the growth of new nerve cells, blood vessels, and support structures in the brain. Approaches such as cell-based therapy, gene therapy, and the administration of growth factors have shown promise in preclinical studies and are currently being investigated in clinical trials. These therapies have the potential to improve tissue repair, functional recovery, and quality of life for stroke survivors.
In addition to these regenerative therapies, other approaches such as rehabilitation, neurostimulation, and bioengineering are also being investigated to improve stroke recovery (Figure 3). Rehabilitation therapies such as physiotherapy, occupational therapy, and speech therapy help stroke survivors regain function and independence [22].
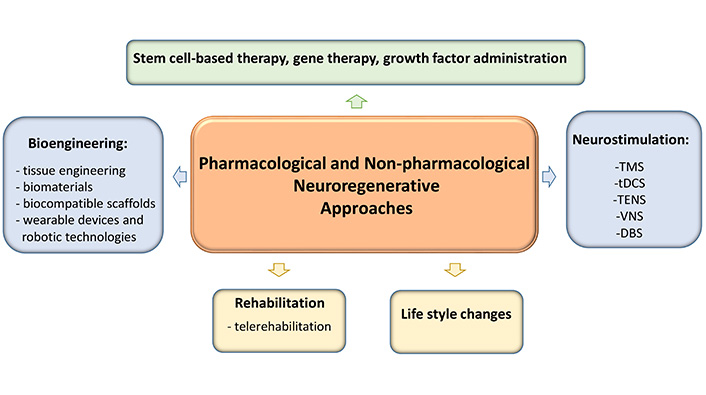
Post-stroke neuroregeneration: pharmacological and non-pharmacological approaches. TMS: transcranial magnetic stimulation; tDCS: transcranial direct current stimulation; TENS: transcutaneous electrical nerve stimulation; VNS: vagus nerve stimulation; DBS: deep brain stimulation
Neurostimulation techniques have been studied and used in stroke rehabilitation to aid motor recovery, promote neural plasticity, and improve cognitive function [125]. Some of the most commonly used techniques are:
Transcranial magnetic stimulation (TMS) uses a magnetic coil to deliver targeted magnetic pulses to specific areas of the brain to modulate neuronal activity. TMS can be used to stimulate the motor cortex and promote motor recovery in stroke patients [126].
In transcranial direct current stimulation (tDCS), a weak electrical current is applied to the scalp to modulate neuronal excitability. Studies have shown that tDCS can improve motor recovery in stroke patients by promoting neuronal plasticity and facilitating motor learning [127, 128].
Transcutaneous electrical nerve stimulation (TENS) uses electrical stimulation to target nerves and muscles involved in movement. TENS can help to improve muscle strength and motor function in stroke patients [125, 129].
Vagus nerve stimulation (VNS) involves implanting a device that electrically stimulates the vagus nerve in the neck. VNS has been shown to improve motor function and reduce inflammation in stroke patients [125].
In deep brain stimulation (DBS), electrodes are implanted in specific areas of the brain to deliver electrical stimulation and modulate neuronal activity. DBS has shown promising results in improving motor function in stroke patients [125]. However, further research is still needed to determine the optimal parameters and protocols for each technique and their long-term effects on post-stroke recovery.
Bioengineering approaches such as tissue engineering and biomaterials can provide scaffolds and growth factors to support tissue repair and regeneration in the brain after stroke [130]. Tissue engineering has shown great potential in the treatment of stroke, as it offers new possibilities for the repair and regeneration of damaged brain tissue. One of the most important approaches in tissue engineering for stroke is the use of bioengineering techniques to develop biocompatible scaffolds or matrices that can support the growth and differentiation of nerve cells [131].
These scaffolds can be engineered to mimic the structure and function of native brain tissue and provide a supportive environment for the regeneration of neurons and blood vessels in the damaged area [132]. Additionally, bioactive molecules and growth factors can be incorporated into the scaffolds to further promote cell survival, growth, and differentiation [133]. Furthermore, stem cell-based therapies can also be combined with tissue engineering techniques to improve the regenerative potential of damaged brain tissue [132]. Ongoing research in this field focuses on optimising scaffold design, incorporating bioactive molecules, and improving stem cell therapies to further improve the effectiveness of tissue engineering in stroke treatment. In addition, researchers are exploring the use of biomaterials to deliver drugs or cells into the brain to promote tissue repair and regeneration after a stroke [133].
Wearable devices and robotic technologies are being developed to support rehabilitation after a stroke. These devices help people regain their mobility, improve their motor skills, and facilitate activities of daily living after a stroke [134].
Computational modelling and simulation techniques are used to analyse complex biological processes in the brain and predict the outcomes of stroke therapies. These approaches help researchers to understand the underlying mechanisms of stroke and develop targeted treatment strategies [135].
By integrating engineering principles into neuroscience and clinical practise, researchers can develop innovative solutions to address the challenges of stroke care and rehabilitation. Further research and clinical trials are needed to validate the safety and efficacy of regenerative therapies and make them usable for stroke patients.
Conclusions
Overall, the treatment of ischemic stroke is a complex and multifaceted process that requires a combination of acute measures as well as longer-term strategies to promote brain repair and recovery. The development of more specific and targeted therapeutics is one of the prospects for the future.
Abbreviations
ATF6: | activating transcription factor 6 |
ATP: | adenosine triphosphate |
BBB: | blood-brain barrier |
CHOP: | C/EBP homologous protein |
CNS: | central nervous system |
CT: | computed tomography |
DBS: | deep brain stimulation |
DTI: | diffusion tensor imaging |
ER: | endoplasmic reticulum |
fMRI: | functional magnetic resonance imaging |
iPSCs: | induced pluripotent stem cells |
IRE1: | inositol-requiring enzyme 1 |
MMPs: | matrix metalloproteinases |
MRI: | magnetic resonance imaging |
MSCs: | mesenchymal stem cells |
MSU: | mobile stroke units |
NETs: | neutrophil extracellular traps |
NF-κB: | nuclear factor kappa B |
NMDA: | N-methyl-D-aspartate |
NSCs: | neural stem cells |
NVU: | neurovascular unit |
OMM: | outer mitochondrial membrane |
PERK: | protein kinase R-like endoplasmic reticulum kinase |
ROS: | reactive oxygen species |
tDCS: | transcranial direct current stimulation |
TENS: | transcutaneous electrical nerve stimulation |
TMS: | transcranial magnetic stimulation |
tPA: | tissue plasminogen activator |
UPR: | unfolded protein response |
VEGF: | vascular endothelial growth factor |
VNS: | vagus nerve stimulation |
XBP1: | X-box binding protein 1 |
Declarations
Acknowledgments
To all my co-workers during the past two decades in the field of cerebral ischemia.
Author contributions
LR: Conceptualization, Investigation, Writing—original draft, Writing—review & editing, Validation.
Conflicts of interest
The author declares no conflict of interest.
Ethical approval
Not applicable.
Consent to participate
Not applicable.
Consent to publication
Not applicable.
Availability of data and materials
Not applicable.
Funding
Not applicable.
Copyright
© The Author(s) 2024.